GCSE Physics – Particle model of matter
The Kinetic Theory of Matter
The Kinetic Theory of Matter is a fascinating concept that helps explain why different materials behave as they do. It helps us understand everything from why ice melts to why balloons deflate.
The Basics of the Kinetic Theory
The Kinetic Theory of Matter tells us three key things:
- All matter is made up of tiny particles. These particles could be atoms, molecules, or ions.
- These particles are always in motion – moving around, vibrating, or spinning.
- The higher the temperature, the faster the particles move.
States of Matter
The Kinetic Theory also helps explain the three states of matter: solids, liquids, and gases.
- Solids. In a solid, the particles are packed together tightly in a regular pattern and can only vibrate in place. Think of it like a classroom where all the students (particles) are sitting at their desks (fixed positions) and can only wiggle around in their seats.
- Liquids. In a liquid, the particles are still close together, but they can slide past each other. This is why liquids can flow. It’s like a busy train station where people (particles) are moving in all directions, occasionally bumping into each other.
- Gases. In a gas, the particles are much further apart and move freely. This is why gases fill their containers and are easy to compress. Imagine being in a large park with friends (particles). You all have plenty of space to run around freely!
Energy and Particle Motion
Energy, in the form of heat, has a direct impact on the motion of particles. If you heat a solid, the particles gain energy and start to vibrate faster. If they gain enough energy, the solid will melt and become a liquid. This is why ice melts into water when you leave it out of the freezer. The room temperature gives energy to the ice, making the water molecules vibrate faster and break apart from their fixed positions, turning the ice into a liquid.
Particle Collisions and Pressure
The particles in a gas are in constant, random motion. When they collide with each other or the walls of their container, they exert a force. This force over a certain area is known as pressure. Think of a balloon: when you inflate it, the air particles inside the balloon are moving quickly and constantly hitting the inner walls of the balloon, causing it to expand. That’s the power of particle motion!
Understanding the Kinetic Theory of Matter is like gaining superpowers. It lets you see into the hidden world of atoms and molecules, and explains why matter behaves as it does. And remember, just like the puppies in our earlier example, particles in all states of matter are always moving – whether slowly and calmly or quickly and energetically!
States of Matter and Particle Arrangement
Understanding the states of matter is like unlocking the secrets of the universe – on a tiny, microscopic scale! This is where we look at how particles are arranged in solids, liquids, and gases. Let’s explore!
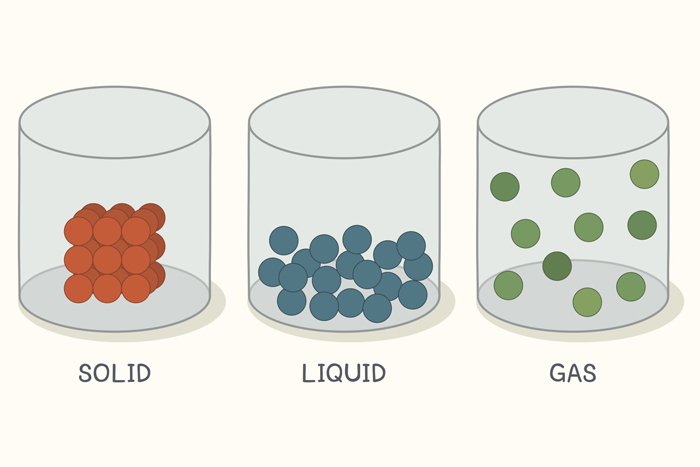
Solid State
In a solid state, particles are closely packed together in a fixed, regular pattern. Imagine a perfectly organised classroom where all students (our particles) are sitting at their desks. They can’t wander around – instead, they vibrate slightly at their fixed positions. This arrangement is why solids retain their shape and volume, and why they’re generally not compressible.
A good example is a diamond. Its particles are rigidly held together in a tight, 3D pattern. The strength of these bonds is what makes diamonds one of the hardest substances known to humans!
Liquid State
In the liquid state, particles still stay close together, but they have more freedom to move around each other, leading to an irregular, dynamic arrangement. This is like a school dance, where students (particles) are mingling with each other, moving around freely but still staying in the same room.
This explains why liquids can flow and take the shape of their container while keeping a constant volume. Imagine pouring water into a glass, a bowl, or a teapot – no matter the container’s shape, the water adapts!
Gas State
In the gas state, particles are much further apart than in solids or liquids and move freely in all directions. This is like kids in a playground – they’re all over the place, running freely and spreading out as much as possible!
Because of this, gases not only take the shape of their container but also expand to fill its entire volume. You’ve likely seen this when you pump air into a bicycle tire – the air (a gas) fills up the whole tire, not just part of it.
How Temperature Influences States of Matter
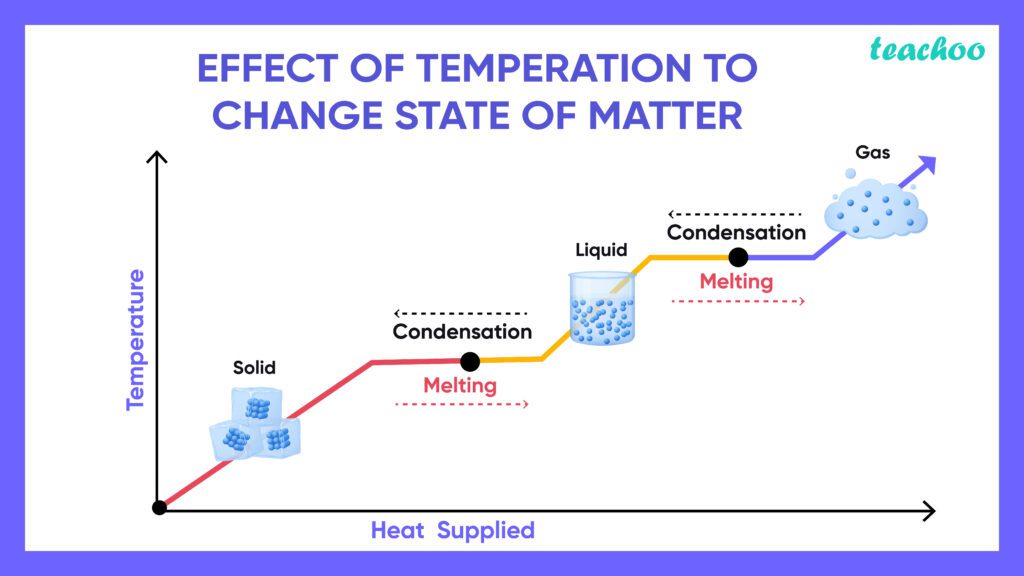
Temperature plays a crucial role in determining the state of matter by influencing the energy of the particles. When you heat a solid like ice, its particles gain energy and start to vibrate more rapidly. If they gain enough energy to overcome the bonds holding them together, they start to slide past each other and the solid becomes a liquid – your ice melts into water!
With even more heat, the water particles gain more energy, moving faster and faster until they can escape the liquid and become a gas – water turns into water vapor, and you see this process when you boil a kettle for tea!
So remember, whether we’re talking about students in a classroom, a school dance, or kids in a playground, the way particles arrange themselves in solids, liquids, and gases shapes our world at the most fundamental level. It’s all about how much freedom the particles have to move around!
Understanding Density
Have you ever wondered why some objects float in water while others sink? Or why a helium balloon rises in the air? The answer lies in a fascinating property of matter called density. Let’s get to know more about it!
What is Density?
Density is a measure of how much mass is packed into a given volume. Think of it like a party. If you have a small room packed with lots of people, the party is very dense. But if you have the same number of people in a large hall, the party is less dense.
In scientific terms, we calculate density using the formula: density = mass/volume. It tells us how much “stuff” (the mass) is squeezed into a certain amount of space (the volume).
Density and States of Matter
Different states of matter generally have different densities. Here’s the usual order (from highest to lowest density): solids, liquids, gases.
Solids: Since the particles in a solid are closely packed together, solids generally have the highest density.
Liquids: Particles in a liquid are close but they can move past each other, which typically makes liquids less dense than solids.
Gases: Particles in a gas are far apart and move freely, which usually results in the lowest density.
Density and Buoyancy
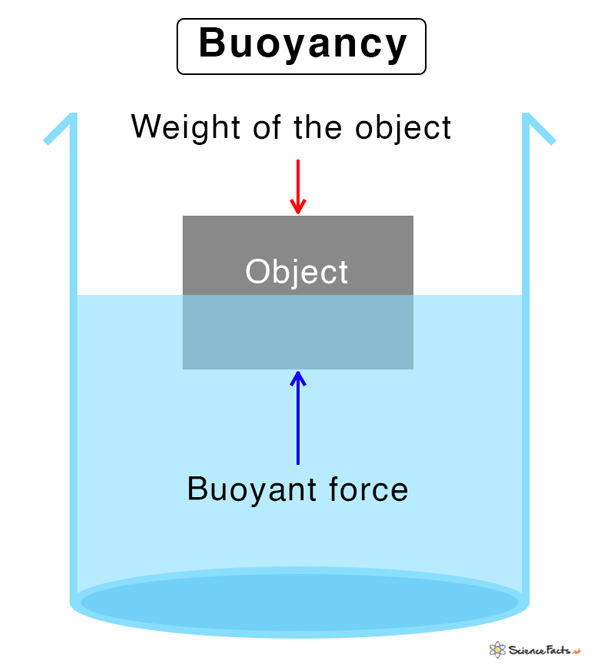
Density is also the key to understanding buoyancy – why some things float and others sink. If an object is less dense than the fluid it’s placed in, it will float. If it’s denser, it will sink.
For example, a log of wood floats in water because its density is less than that of water. On the other hand, a stone sinks because its density is greater than water’s. And a helium balloon rises in the air because helium is less dense than the surrounding air!
Experimenting with Density
One fun way to visualise density is by making a density column using liquids of different densities. If you carefully layer honey, dish soap, water, oil, and alcohol in a clear glass, they won’t mix, forming layers from most dense at the bottom to least dense at the top. Give it a try!
Understanding density not only explains everyday phenomena like why things sink or float, but it also helps scientists predict how substances will behave under different conditions. From designing ships to float on water, to making lighter-than-air aircraft, understanding density is crucial!
Law of Conservation of Mass
Ever wondered why you can’t make something from nothing, or turn something into nothing? It’s all thanks to the Law of Conservation of Mass. Let’s dive into what this law means and why it’s so crucial in our understanding of the physical world.
What is the Law of Conservation of Mass?
In simple terms, the Law of Conservation of Mass states that matter cannot be created or destroyed in a closed system. This means that the total mass of all the substances involved in a chemical reaction or a physical change stays the same before and after the process.
Picture it like this: Suppose you have a bag of Lego bricks. You can build a castle, a car, a spaceship, or a dragon using those bricks. You can dismantle them and build something new over and over again. But no matter what you create, you’ll still have the same number of Lego bricks. You can’t create new bricks out of thin air or make some disappear into nothingness.
Conservation of Mass in Chemical Reactions
In a chemical reaction, the Law of Conservation of Mass is evident in the balanced chemical equation. The number and type of atoms in the reactants (substances before the reaction) must equal the number and type of atoms in the products (substances after the reaction).
Let’s take a simple chemical reaction like the combustion of methane (natural gas):
CH₄ (methane) + 2O₂ (oxygen) → CO₂ (carbon dioxide) + 2H₂O (water)
If you count the atoms on each side of the equation, you’ll find that they’re equal: one carbon, four hydrogen, and four oxygen atoms. This is a perfect illustration of the Law of Conservation of Mass.
Conservation of Mass During Changes of State
The Law of Conservation of Mass also applies when substances change state, like when ice melts into water or water boils into steam. Even though the physical appearance and properties of the substance change, its mass remains the same.
If you were to weigh a block of ice and then melt it and weigh the resulting water, you’d find the mass to be the same. The water molecules in the ice didn’t disappear or multiply; they simply rearranged to change from solid to liquid.
Importance of the Law of Conservation of Mass
This law is fundamental to the study of physics and chemistry. It helps us make predictions in chemical reactions, understand energy transfer, and even balance chemical equations. It’s one of the basic laws that govern how the universe works!
So next time you see a magic trick that seems to create or vanish objects, remember the Law of Conservation of Mass. The “magic” is just clever misdirection, not a violation of the fundamental laws of science!
Heating and Cooling Graphs
Heating and cooling graphs are like windows into the world of particles. They provide a visual way of understanding how substances change state as they gain or lose energy. So let’s turn up the heat (or cool things down!) and dive into this topic.
Understanding Heating and Cooling Graphs
Heating and cooling graphs usually show temperature on the vertical axis and time (or heat input/output) on the horizontal axis. As heat is added or removed, the temperature of a substance changes, and these changes can be plotted on the graph.
Here’s the exciting part: These graphs also show when a substance changes its state – from solid to liquid (melting), liquid to gas (boiling), or the reverse during cooling.
What Happens During Heating?
Imagine you start with a solid (like ice) and begin to heat it.
Stage 1 – Solid Heating
Initially, the temperature of the solid rises as the particles vibrate more and more due to the increase in energy. This is shown by an upward sloping line on the graph.
Stage 2 – Melting
Once the substance reaches its melting point, it begins to change from a solid to a liquid. During this phase, the temperature doesn’t increase even though you’re still adding heat. The extra energy is used to break the bonds between the particles. On the graph, this appears as a flat line.
Stage 3 – Liquid Heating
After all the solid has melted into liquid, the temperature begins to rise again as the liquid particles gain more energy and move faster. This is another upward sloping line on the graph.
Stage 4 – Boiling
Once the boiling point is reached, the liquid starts changing into a gas. Again, even though heat is being added, the temperature remains constant as the energy is used to break the bonds completely. This forms another flat line on the graph.
Stage 5 – Gas Heating
After all the liquid has vaporised into a gas, the temperature starts to rise once again with the increase in heat, which is shown as an upward line on the graph.
What Happens During Cooling?
The cooling process is just the reverse of heating. Instead of adding heat, you’re taking it away. The substance goes from gas to liquid (condensation), then from liquid to solid (freezing), each time the temperature staying constant as the state change occurs.
The Power of Heating and Cooling Graphs
These graphs are valuable tools for scientists and engineers. They help us understand how different substances behave under various conditions, guiding everything from weather forecasting to the design of engines and refrigeration systems.
So remember, every time you boil water for a cup of tea or freeze ice cubes for a refreshing drink, you’re experiencing the science that heating and cooling graphs describe!
Specific Heat Capacity
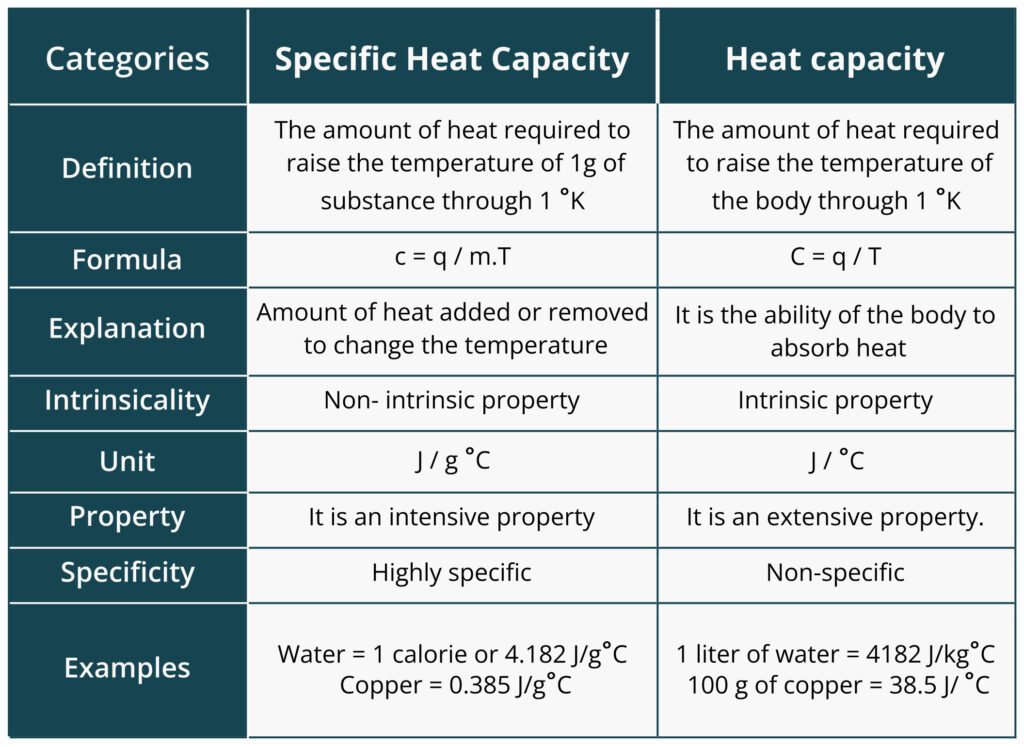
If you’ve ever noticed that different substances heat up or cool down at different rates, you’ve encountered the concept of specific heat capacity. This principle underlies everything from why beaches feel hot in the summer to how our bodies maintain a stable temperature. So, let’s delve into what specific heat capacity is all about!
What is Specific Heat Capacity?
Specific heat capacity (often just called specific heat) is a property that tells us how much heat energy is needed to raise the temperature of a certain amount of a substance by a certain amount.
In scientific terms, it’s defined as the amount of heat energy required to raise the temperature of 1 kilogram of a substance by 1 degree Celsius (or Kelvin). It’s usually represented by the symbol ‘c’ and measured in joules per kilogram per degree Celsius (J/kg°C).
Specific Heat and Different Substances
Different substances have different specific heat capacities. This means they require different amounts of heat energy to increase their temperatures.
For example, water has a high specific heat capacity, which means it takes a lot of heat energy to raise its temperature. This is why the water in a swimming pool doesn’t instantly warm up on a hot day. On the other hand, metals like iron or aluminium have lower specific heat capacities, which is why a metal spoon in a hot pot can quickly become too hot to touch!
Practical Applications of Specific Heat Capacity
Understanding specific heat capacity has many practical applications. For instance, engineers use this concept to design heating and cooling systems for buildings and vehicles. Chefs use it to select the right cooking methods and tools – ever wondered why many pots and pans are made of metals?
Another fun fact is that our planet’s large bodies of water, with their high specific heat, help regulate Earth’s climate. They absorb heat from the sun during the day and release it slowly at night, preventing extreme temperature swings.
Calculating Heat Energy
You can calculate the heat energy (Q) added or removed from a substance using the formula:
Q = mcΔT
where ‘m’ is the mass of the substance, ‘c’ is the specific heat capacity, and ‘ΔT’ is the change in temperature. This equation allows scientists and engineers to precisely control temperature changes in various applications, from industrial processes to household appliances.
Specific Latent Heat
Ever wondered why ice takes time to melt even in a hot room or why boiling water stays at the same temperature for a while? The answer lies in the concept of specific latent heat. This principle helps explain many everyday phenomena and is a key part of understanding how heat energy interacts with matter.
What is Specific Latent Heat?
Specific latent heat is the amount of heat energy required to change the state of a substance without changing its temperature. The word ‘latent’ comes from a Latin word that means ‘hidden’, because this heat energy is ‘hidden’ as it does not cause a temperature change.
There are two types of specific latent heat:
- Specific latent heat of fusion is the heat energy needed to change a substance from solid to liquid (or vice versa) at its melting point.
- Specific latent heat of vaporisation is the heat energy needed to change a substance from liquid to gas (or vice versa) at its boiling point.
Specific Latent Heat and Different Substances
Just like specific heat capacity, different substances also have different specific latent heats. This means they require different amounts of heat energy to change their states.
For example, water has a high specific latent heat of fusion, so it takes a lot of heat energy to melt ice into water. This is why ice cubes don’t melt immediately even in a warm drink!
Practical Applications of Specific Latent Heat
Understanding specific latent heat has many practical applications.
In our homes, it explains why food remains at boiling temperature until it’s fully cooked, as the heat energy is being used to change water from liquid to steam.
In the environment, the high specific latent heat of vaporization for water helps moderate Earth’s climate. Large amounts of solar energy are absorbed by the oceans, melting ice and evaporating water, which cools the surface and warms the atmosphere.
Calculating Heat Energy During State Changes
The heat energy (Q) involved in a state change can be calculated using the formula:
Q = mL
where ‘m’ is the mass of the substance and ‘L’ is the specific latent heat of fusion or vaporization. This equation allows scientists and engineers to design systems that control or utilize state changes, from refrigeration systems to power plants.
In short, specific latent heat is a fascinating concept that reveals how heat energy is hidden in the changes of state of substances. From the ice in your drink to the steam from a boiling kettle, it’s a part of our daily life!
Relationship between Gas Pressure, Volume, and Temperature
Gases are all around us – in the air we breathe, the balloons we inflate, and even the fizzy drinks we enjoy. But did you know gases behave differently under different conditions? Understanding these behaviours can be intriguing, and that’s where we explore the relationship between gas pressure, volume, and temperature.
The Gas Laws
Three fundamental laws – Boyle’s Law, Charles’s Law, and Gay-Lussac’s Law – describe how gases behave under different conditions:
1. Boyle’s Law states that the pressure of a gas is inversely proportional to its volume when the temperature is kept constant. Imagine you’re inflating a balloon. As you add more air (increasing pressure), the balloon expands (the volume increases). Now if you squeeze the balloon (decreasing the volume), the pressure inside the balloon increases.
2. Charles’s Law states that the volume of a gas is directly proportional to its temperature when the pressure is kept constant. Consider a gas-filled balloon on a hot day. As the day gets hotter (increasing temperature), the balloon gets bigger (the volume increases).
3. Gay-Lussac’s Law states that the pressure of a gas is directly proportional to its temperature when the volume is kept constant. Imagine a sealed can of soda. If you shake it or leave it in the sun (increasing the temperature), the pressure inside the can also increases.
The Ideal Gas Law
While Boyle’s, Charles’s, and Gay-Lussac’s laws each hold true under specific conditions, the Ideal Gas Law combines these concepts to describe how gases behave under any conditions:
PV = nRT
Where P is pressure, V is volume, n is the number of moles of gas, R is the universal gas constant, and T is temperature. This equation shows how pressure, volume, and temperature are interconnected for any given amount of gas.
Practical Applications
Understanding the relationship between gas pressure, volume, and temperature has many real-life applications.
For instance, weather balloons are designed with Charles’s Law in mind. As they rise through the atmosphere, the outside air pressure decreases, allowing the balloon to expand.
Similarly, scuba divers must consider Boyle’s Law when ascending from deep dives. As they ascend and pressure decreases, the volume of gases in their lungs and bloodstream can increase, leading to potential health hazards.
The principles that govern the behaviour of gases help us in everything from predicting weather changes, to designing engines, to understanding how we breathe. They show us that the air around us isn’t just empty space – it’s a dynamic mixture that responds and adapts to the world around it!
Changes in States of Matter
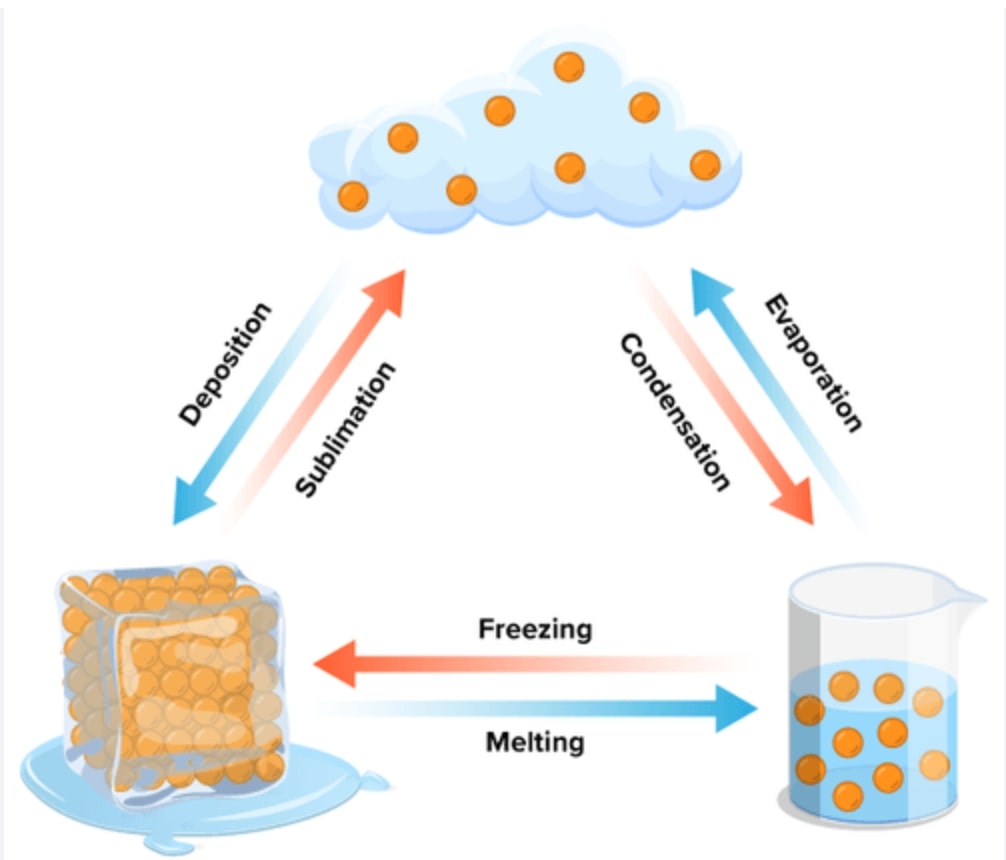
Everything around us is made up of matter, which exists in three primary states – solid, liquid, and gas. But did you know that these states aren’t permanent and can change under different conditions? Let’s explore how and why these changes in states of matter occur.
What are State Changes?
A change of state, also called a phase change, occurs when matter transforms from one state – solid, liquid, or gas – to another. This transformation is usually the result of adding or removing heat, but changes in pressure can also induce state changes.
Types of State Changes
There are six primary state changes:
1. Melting
When a solid absorbs heat, its particles gain energy and start to move more freely, transitioning the solid into a liquid. This process is called melting. The temperature at which a solid melts is known as its melting point. For example, ice melts into water at 0 degrees Celsius under standard pressure conditions.
2. Freezing
Freezing is the opposite of melting. When a liquid loses heat, its particles slow down and form a structured pattern, becoming a solid. The temperature at which this happens is called the freezing point.
3. Evaporation
If a liquid gains enough heat, it turns into a gas, a process called evaporation. You see this when water in a pot starts boiling and forms steam.
4. Condensation
The reverse of evaporation is condensation. When a gas loses heat, it turns back into a liquid. This is what happens when steam cools down to form water droplets on a cold surface.
5. Sublimation
In some cases, a solid can transform directly into a gas without becoming a liquid first. This process is called sublimation. Dry ice, which is solid carbon dioxide, sublimates at room temperature.
6. Deposition
Deposition, or desublimation, is the reverse of sublimation. It’s when a gas transforms directly into a solid, skipping the liquid phase. This is how frost forms on a cold window.
Conservation of Mass during State Changes
One important principle to remember is that during any of these changes, the mass of the substance remains the same. This is called the Law of Conservation of Mass. For example, the total mass of water is the same whether it’s in the form of ice, liquid water, or steam.
The Fascinating Dance of Particles
State changes are like a dance of particles, moving from the structured patterns of solids to the free flow of gases and back again. By understanding these changes, we can explain everything from why we need to defrost our freezers, to how the water cycle affects the Earth’s climate. It’s another way that science helps us make sense of the world around us!
Absolute Zero
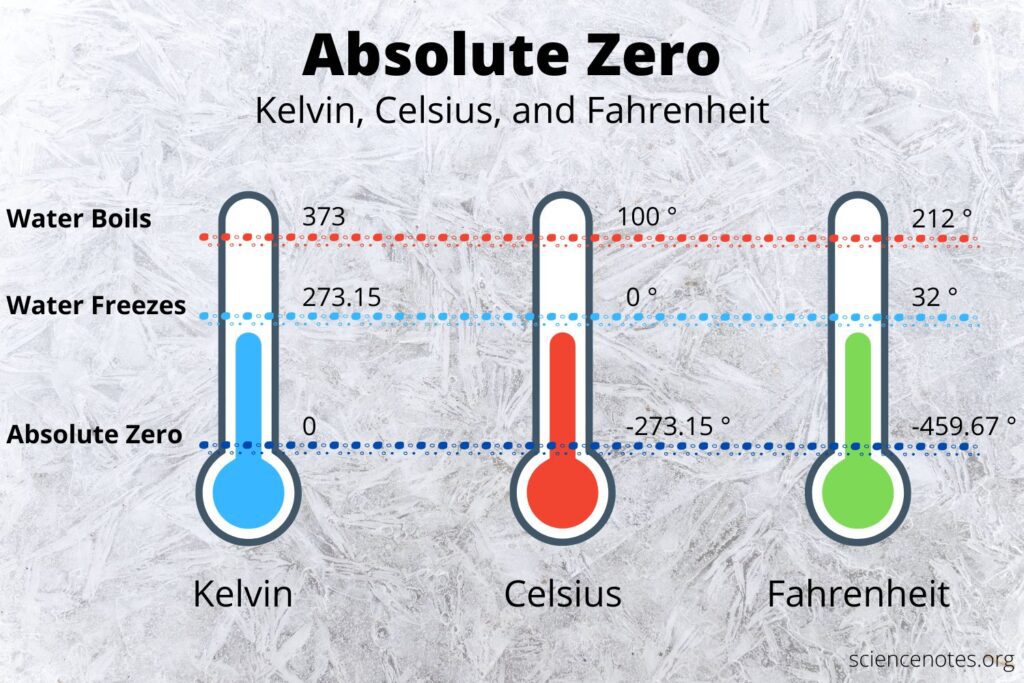
Imagine the coldest place you can. Now, think even colder. When scientists talk about ‘absolute zero’, they’re referring to the lowest possible temperature – a point where all molecular motion stops. Let’s dig into what absolute zero is and why it’s important.
What is Absolute Zero?
Absolute zero is the theoretical temperature at which substances have minimum internal energy. In other words, at absolute zero, particles stop moving and have zero kinetic energy. It’s like the ultimate freeze!
In the Celsius scale, absolute zero is defined as -273.15 degrees Celsius. However, for scientific purposes, we often use the Kelvin scale, which starts at absolute zero (0 Kelvin).
Absolute Zero and the Third Law of Thermodynamics
The concept of absolute zero is directly related to the Third Law of Thermodynamics, which states that the entropy of a perfect crystal is zero at absolute zero. Entropy is a measure of disorder or randomness. So, at absolute zero, a perfect crystal would have perfect order.
Can We Reach Absolute Zero?
So, you might be wondering, can we ever reach absolute zero? The answer is fascinatingly, no. According to the laws of quantum mechanics, particles have a property known as zero-point energy, which keeps them in constant, minimal motion. This means we can never remove all the energy from a system or reach absolute zero temperature.
However, scientists can get remarkably close. Through advanced cooling techniques, scientists have reached temperatures just fractions of a Kelvin above absolute zero. These extreme conditions are used to study quantum phenomena and other scientific applications.
Practical Implications of Absolute Zero
Absolute zero might seem like an abstract concept, but it has many practical implications. For example, the Kelvin temperature scale, starting from absolute zero, is used in scientific research and industries.
Additionally, understanding absolute zero helps us to comprehend the theoretical limits of cooling, explore states of matter at ultra-low temperatures, and inform fields as diverse as materials science, quantum computing, and cosmology.
In conclusion, while absolute zero is a temperature we can’t technically reach, it’s a concept that holds fascinating implications for our understanding of the universe.
The Ideal Gas Law
While we live in a world full of complex gases, scientists often simplify things using the concept of an ‘ideal gas’. This is a hypothetical gas that perfectly follows a mathematical relationship known as the Ideal Gas Law. Let’s dive into what this law is and why it’s so important!
What is the Ideal Gas Law?
The Ideal Gas Law is a fundamental principle in the study of physical chemistry and thermodynamics. It describes how gases behave under varying conditions of pressure, volume, and temperature.
The law is expressed as the equation:
PV = nRT
Where:
- P is the pressure of the gas,
- V is the volume occupied by the gas,
- n is the number of moles of the gas,
- R is the universal gas constant,
- T is the absolute temperature of the gas (measured in Kelvin).
This equation tells us that for a given amount of gas at a constant temperature, the product of pressure and volume is constant.
Understanding the Ideal Gas Law
The Ideal Gas Law allows us to predict how a gas will behave under different conditions. For instance, if you increase the temperature of a gas (while keeping the number of moles and volume constant), the pressure will increase. This is why a car tire can burst if it gets too hot – the increased temperature causes the gas inside the tire to increase pressure.
Similarly, if you decrease the volume of a gas (while keeping the number of moles and temperature constant), the pressure will also increase. This is what happens when you pump more air into a bicycle tire – the volume of the tire remains constant, but the added air (increased moles of gas) increases the pressure inside the tire.
Ideal vs Real Gases
In reality, no gas behaves perfectly ideally. The Ideal Gas Law is a simplification that assumes gas particles do not interact with each other and occupy no space. In the real world, gas particles do have volume and do interact.
However, many gases behave closely to ideal at near room temperature and low pressure, making the Ideal Gas Law a very useful approximation for many scientific and engineering calculations.
Practical Applications of the Ideal Gas Law
The Ideal Gas Law has a wide range of practical applications. It is used in engineering to design and understand systems in which gases are used, such as engines and refrigeration systems. It’s also used in weather science to help predict and understand atmospheric changes.
In short, the Ideal Gas Law is a cornerstone of our understanding of gases. By grasping it, we can make sense of the complex dance of molecules that fill our universe!
Brownian Motion
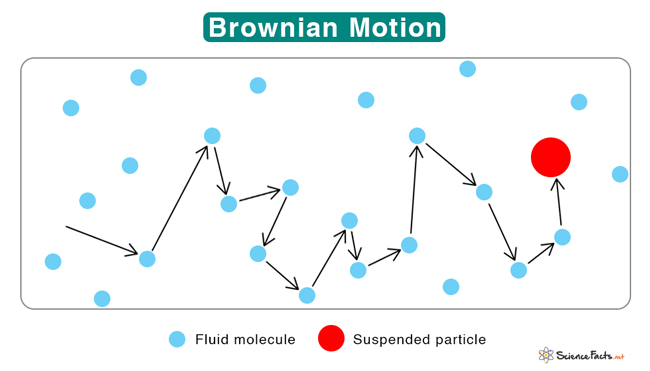
You might think that particles in a gas or liquid just sit quietly, but if you look closely enough, you’ll see they’re in a constant, jittery dance. This random motion is called Brownian Motion, and it’s a fundamental concept in physics and chemistry.
What is Brownian Motion?
Brownian motion is the random, erratic movement of tiny particles suspended in a fluid (liquid or gas). This motion is caused by the continuous, random collisions these particles experience with the faster-moving molecules of the fluid.
The concept is named after the Scottish botanist Robert Brown. In 1827, while observing pollen grains floating in water under a microscope, he noticed that the grains moved about in a seemingly random, haphazard manner.
How Does Brownian Motion Work?
Imagine a small particle, like a speck of dust, floating in air. This particle is constantly being hit by the air molecules around it. Since these collisions come from all directions and with different strengths, they push the dust particle in a random, erratic path. This is Brownian motion in action.
It’s important to note that while the motion appears random, it is not uncaused – it’s a result of the random, individual collisions with the fluid’s molecules.
Brownian Motion and the Particle Theory of Matter
Brownian motion provides a visual proof for the particle theory of matter and the existence of atoms and molecules. Robert Brown’s observations supported the idea that matter is made up of small, discrete particles (like the water molecules that were colliding with the pollen grains).
Applications of Brownian Motion
Brownian motion has a range of practical applications and implications. For instance, it’s essential in diffusion processes, like how a drop of dye spreads in water. It’s also used in mathematical modeling of random phenomena, found in fields as diverse as finance, ecology, and computer science.
Moreover, understanding Brownian motion can help us design tiny machines and navigate the world on the microscopic scale, a key aspect of nanotechnology.
In conclusion, Brownian motion is a fascinating manifestation of the molecular world in action. It brings to life the abstract concept of atoms and molecules and helps us understand and predict the behavior of particles in the microscopic world!
Internal Energy
Energy is a fundamental concept in physics, and it can be found in various forms. One such form is the internal energy, a term that describes the energy associated with the microscopic components of a system. Let’s explore what internal energy is and why it’s so important.
What is Internal Energy?
Internal energy is the total energy stored in the molecules of a substance. It includes:
1. Kinetic Energy: The energy of the particles due to their motion. This includes both translational (movement from one place to another), rotational (spinning), and vibrational (back-and-forth) motion.
2. Potential Energy: The energy of the particles due to their positions or configurations. This is often related to the intermolecular forces between particles.
So, when we talk about internal energy, we’re referring to the sum of all kinetic and potential energy of all particles in a system.
How is Internal Energy Changed?
There are two primary ways the internal energy of a system can be changed:
1. Heat Transfer: If heat is added to a system, it increases the kinetic energy of the particles, thereby increasing the internal energy. Conversely, if heat is removed, the internal energy decreases.
2. Work: Work can also change a system’s internal energy. If work is done on a system (like compressing a gas), it increases the system’s internal energy. If the system does work (like expanding a gas), it loses internal energy.
Internal Energy and the First Law of Thermodynamics
The concept of internal energy is central to the First Law of Thermodynamics, which states that the change in internal energy of a system is equal to the heat added to the system minus the work done by the system.
In a formula, it’s often written as:
ΔU = Q – W
Where ΔU is the change in internal energy, Q is the heat added to the system, and W is the work done by the system.
Practical Applications of Internal Energy
Understanding internal energy has many practical implications. For example, it’s crucial in the study and design of heat engines and refrigerators. It’s also important in understanding phase changes, like why adding heat to ice first melts it (a change in potential energy) before raising its temperature (a change in kinetic energy).
In essence, internal energy helps us comprehend the unseen, microscopic dance of molecules and how energy moves and changes forms at the smallest scales!
Energy Transfer and Changes of State
Ever wondered why an ice cube melts when left out of the freezer or why water boils when heated? These changes of state are all about energy transfer. Let’s take a closer look at how energy plays a role in these fascinating processes.
Energy and Changes of State
When a substance changes state, like from a solid to a liquid or from a liquid to a gas, it’s because energy is being added to or removed from the substance. This energy is used to change the motion of the particles and the bonding between them, which in turn changes the state of the substance.
Let’s break down how this works for the main state changes:
1. Melting (Solid to Liquid): When a solid is heated, the energy from the heat source is transferred to the particles in the solid, causing them to move more rapidly. This increased motion weakens the bonds between the particles until they can move past each other, turning the solid into a liquid.
2. Evaporation or Boiling (Liquid to Gas): Adding more energy to a liquid increases the motion of the particles even further. Eventually, the particles move so quickly and with such energy that they break free from their bonds entirely, becoming a gas.
3. Freezing (Liquid to Solid): When energy is removed from a liquid (by cooling it, for example), the particles slow down. As they move less, the bonds between them strengthen, and they arrange themselves into a structured pattern, becoming a solid.
4. Condensation (Gas to Liquid): If a gas loses energy (typically by cooling), the particles slow down. As their motion decreases, the particles can form bonds and become a liquid.
Energy Transfer is Not Always Obvious
One interesting point to remember is that when energy is being used to change the state of a substance, it might not cause a change in temperature. This is because the energy is being used to break or form bonds between particles, rather than increase their motion.
This is why, for example, ice melts at 0 degrees Celsius, and water boils at 100 degrees Celsius. Even though heat is being added in both cases, the temperature doesn’t rise above these points until the change of state is complete.
Conservation of Energy
Throughout all these processes, the principle of conservation of energy holds true. The energy doesn’t disappear; it just changes form, either into the kinetic energy of the particles (raising the temperature) or into potential energy (changing the bonding between particles).
By understanding how energy transfer drives changes of state, we can better understand many of the physical changes we see in the world around us, from the water cycle that drives our weather, to the workings of our refrigerators and stoves!
Diffusion
When you spray perfume in a room, it doesn’t stay in one spot, does it? After a while, the fragrance spreads throughout the room. This spreading process, called diffusion, is a fundamental concept in physics and chemistry. Let’s get into the details!
What is Diffusion?
Diffusion is the process by which particles spread out from an area of high concentration to an area of low concentration until they are evenly distributed. This happens because particles are constantly moving and collide with each other, spreading out to fill all available space.
How Does Diffusion Work?
Think about a drop of food colouring in a glass of water. At first, the colouring is concentrated in one area. But the molecules of the colouring are constantly moving, and so are the water molecules. As they collide with each other, the food colouring molecules spread out until they’re evenly distributed in the water. That’s diffusion!
Importantly, diffusion doesn’t require any external energy. It happens due to the natural, random motion of particles, which is part of the kinetic theory of matter we talked about earlier.
Factors Affecting the Rate of Diffusion
Several factors can affect how quickly diffusion occurs:
1. Concentration Gradient: This is the difference in concentration between two areas. A higher concentration gradient (a bigger difference) will result in faster diffusion.
2. Temperature: As temperature increases, particles move faster, which also speeds up diffusion.
3. Size of Particles: Smaller particles move more quickly and therefore diffuse faster.
4. Medium: Diffusion happens fastest in gases (where particles are far apart and can move freely) and slowest in solids (where particles are close together and can’t move as much).
Diffusion in Our Lives
Diffusion is everywhere! It’s how the smell of baking cookies wafts through your house, or how oxygen moves from your lungs into your bloodstream. It’s also a crucial process in chemical reactions and industrial processes, such as the spreading of pollutants in air or water.
So, diffusion isn’t just an abstract concept in physics, but a fundamental process that shapes our world and our lives. It’s a testament to the constant motion and interaction of particles, even when things seem perfectly still to our eyes!
Conclusion
GCSE Physics students must study this particle model of matter. The model explains the properties and behaviors of the different states of matter, and it is central to many other ideas in Physics at higher levels. In these ways, students learn to acquire an insight into the subject and develop skills in analysis.
Our concentrated GCSE Physics tutors at Edumentors are there to help students learn these elementary ideas. Individual support, tailored for each student by subject field tutors who are highly experienced and knowledgeable at Edumentors, guides them toward the achievement of their intended academic aims. Be it the particle model of matter or any other physics-related topic you want help with, our capable tutors will guide you in every possible way throughout the learning journey.
A GCSE Physics tutors can make all the difference. Let challenging topics not drag you down when seeking help from Edumentors today to realize your full potential in GCSE Physics.
Test Yourself
What is the particle model of matter?
The particle model of matter is a simple theory that describes the properties and behavior of matter. It posits that all matter is made up of tiny particles which are constantly moving and interacting.
How do the particles differ in solids, liquids, and gases in terms of arrangement and energy?
In solids, particles are closely packed in a fixed, orderly pattern and have low energy. In liquids, particles are still close but can slide past each other, having more energy than in solids. In gases, particles are far apart, move freely and have the most energy.
What is the law of conservation of mass?
The law of conservation of mass states that mass cannot be created or destroyed in a closed system through ordinary physical and chemical changes.
What is the difference between specific heat capacity and specific latent heat?
Specific heat capacity is the amount of energy needed to raise the temperature of 1 kg of a substance by 1°C, without a change in state. Specific latent heat, on the other hand, is the energy required to change the state of 1 kg of a substance without a change in temperature.
How does the motion of molecules in a gas relate to its temperature and pressure?
The motion of molecules in a gas is directly proportional to its temperature. The faster the molecules move, the higher the temperature. Similarly, the faster the molecules move and collide with the walls of its container, the higher the pressure of the gas.
What is absolute zero?
Absolute zero, 0 Kelvin or -273.15°C, is the lowest possible temperature. It’s the point where all particle motion would theoretically stop, and no more heat can be removed from a system.
What is the Ideal Gas Law?
The Ideal Gas Law states that the pressure of a gas times its volume is directly proportional to the number of gas molecules and the temperature. It’s often written as PV = nRT, where P is pressure, V is volume, n is number of moles, R is the universal gas constant, and T is temperature in Kelvin.
What is Brownian motion?
Brownian motion is the random, erratic motion of tiny particles suspended in a fluid (liquid or gas), caused by collisions with the fluid’s molecules. It’s evidence for the kinetic theory of matter.
Explain how the arrangement and movement of particles in a solid differ from those in a gas.
In a solid, particles are closely packed in a fixed, orderly pattern and vibrate around fixed positions. In a gas, particles are far apart, move freely and rapidly in all directions.
How does the kinetic theory of matter explain the process of diffusion?
The kinetic theory of matter explains that particles are in constant, random motion. In diffusion, this motion causes particles to spread from an area of higher concentration to an area of lower concentration until they’re evenly distributed.
Why does the temperature of a substance remain constant during a change of state?
During a change of state, the added or removed energy is used to break or form bonds between particles, not to increase or decrease their kinetic energy. Therefore, the temperature remains constant until the change of state is complete.
Describe the process of condensation in terms of energy transfer.
During condensation, a gas loses energy, typically by cooling. As the particles lose energy, they slow down and can form bonds with each other, changing from a gas to a liquid.
How does an increase in temperature affect the rate of diffusion?
An increase in temperature gives particles more kinetic energy, making them move faster. This increases the rate of collisions and therefore the rate of diffusion.
If a 2 kg piece of metal requires 1000 J of energy to raise its temperature by 1°C, what is its specific heat capacity?
The specific heat capacity is defined as the amount of heat per unit mass required to raise the temperature by 1°C. In this case, it would be 1000 J / (2 kg * 1°C) = 500 J/kg°C.
What does the ideal gas law predict about the behavior of a gas when its volume is decreased while the temperature is held constant?
According to the ideal gas law, if the volume of a gas is decreased while the temperature is held constant, the pressure of the gas will increase.
Explain the concept of absolute zero. What does it signify about the motion of particles?
Absolute zero, 0 Kelvin or -273.15°C, is the theoretical temperature at which particles would stop moving altogether. It signifies the lowest limit of temperature where kinetic energy of particles would be zero.
What is Brownian motion and how does it provide evidence for the kinetic theory of matter?
Brownian motion is the random, erratic motion of tiny particles suspended in a fluid, caused by collisions with the fluid’s molecules. It provides evidence for the kinetic theory of matter as it directly shows the effects of the random and constant motion of particles.
Explain how the internal energy of a system can change.
The internal energy of a system can change by adding or removing energy, often in the form of heat or work. For instance, heating a system will increase the kinetic energy of its particles, thus increasing its internal energy.
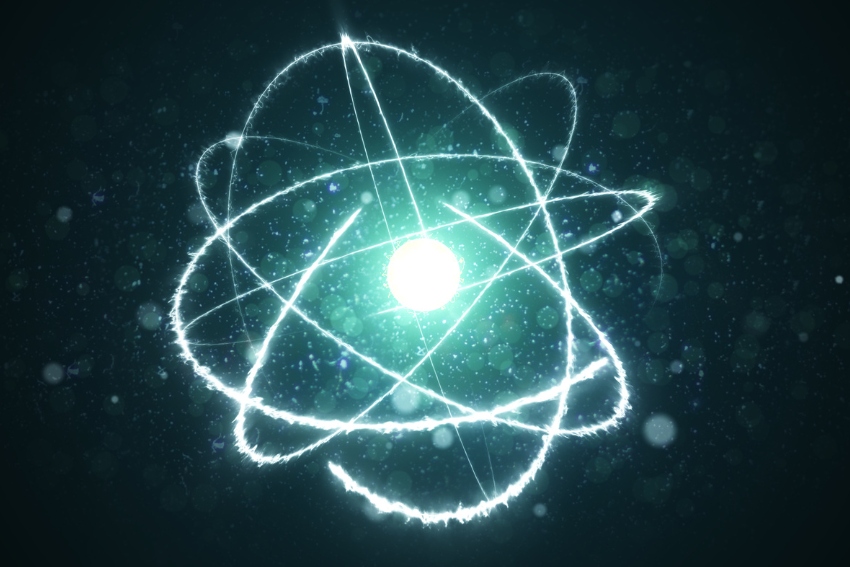