Table of Contents:
- What are the three main types of chemical bonds?
- What is the difference between ionic and covalent bonding?
- What are the properties of ionic compounds?
- How do metallic bonds work?
- What are the properties of covalent compounds?
- What is the difference between simple molecular structures and giant covalent structures?
- What is the structure and property of diamond and graphite?
- What are alloys and how do they differ from pure metals?
- What is the difference between a dot and cross diagram and an electron shell diagram?
- What is the concept of electronegativity in bonding?
- Test Yourself
- Additional Knowledge
- Octet Rule
- VSEPR Theory (Valence Shell Electron Pair Repulsion)
- Pauli Exclusion Principle
- Hund's Rule
- Avogadro's Law
- Metallic Bonding Model
- Ionic Bonding Model
- Covalent Bonding Model
- Conclusion
What are the three main types of chemical bonds?
- Ionic Bonds: Imagine you’re at a party. You see someone holding a bunch of delicious chocolate chip cookies. You really want a cookie. They see this and hand over a cookie willingly. This is a lot like ionic bonding. In ionic bonding, one atom (like you wanting a cookie) really wants an extra electron and another atom (like the person with the cookies) is willing to give up an electron. The atom that loses the electron becomes a positive ion and the one that gains the electron becomes a negative ion. Just like you and the person with the cookies, these two ions are now attracted to each other, forming an ionic bond!
- Covalent Bonds: Now, imagine you’re at the same party, but this time with a massive pizza. You’re nice, so you decide to share it with your friend. You both enjoy the pizza together. This is like a covalent bond! In covalent bonding, two atoms (you and your friend) share electrons (the pizza slices). Both of you get to enjoy the benefits without any of you losing or gaining an electron, just like sharing pizza slices. This happens often between non-metal atoms.
- Metallic Bonds: Think about a bustling city with loads of cars (the electrons) zooming freely along the streets (the metal lattice). This is like metallic bonding, where the electrons are free to move throughout the structure (or the “city”). The ‘sea’ of freely moving electrons gives metals their good electrical and thermal conductivity.
So there you have it! Chemical bonds are like parties with cookies, pizzas, and bustling cities. Whether atoms are taking, sharing, or letting electrons roam free, these interactions form the basis for the materials that make up our world.
What is the difference between ionic and covalent bonding?
Imagine you’re at a game night with friends. Now, let’s think of ionic and covalent bonding as two different types of games you could play: a trading game like Monopoly, and a cooperative game like a team puzzle.
Ionic Bonding: It’s like a trading game, such as Monopoly. In Monopoly, players acquire properties through purchasing or trading – the aim is to collect sets of the same color. Likewise, in ionic bonding, one atom (let’s say it’s like the player who is really good at trading) effectively “trades” its electron(s) to another atom. This creates charged ions: one atom becomes positively charged (having lost an electron) and the other becomes negatively charged (having gained an electron). These oppositely charged ions are attracted to each other, much like players are attracted to lucrative properties in Monopoly.
Covalent Bonding: This is like a cooperative game where everyone works together to complete a puzzle. In a puzzle, you share the pieces and work together to form the complete picture. In covalent bonding, atoms also ‘work together’ by sharing their electron(s) with other atoms. The electrons orbit around both atoms, bonding them together. Nobody wins or loses in this game, much like the team effort in completing a puzzle. This type of bond is common between non-metal atoms.
So, in summary, ionic bonding is like a strategic trading game, where atoms gain or lose electrons, creating charged ions that attract each other. Covalent bonding, on the other hand, is more like a cooperative puzzle game, where atoms share electrons to form a bond. Both are different ways atoms can come together to form molecules, but the rules of the ‘game’ are different!
What are the properties of ionic compounds?
- High Melting and Boiling Points: Imagine a very well-built sand castle. It’s not easy to destroy, right? You need a lot of force (or a very excited dog) to break it apart. Similarly, ionic compounds have high melting and boiling points because the forces between the ions are very strong. It takes a lot of energy (heat) to break these forces and melt or boil the compound.
- Conduct Electricity When Dissolved or Melted: Now, think about a highway with a lot of cars moving along it. When the road is clear, the cars can move freely and quickly. When ionic compounds are dissolved in water or melted, the ions are free to move, much like cars on a highway. These moving ions can carry an electric current, making ionic compounds good conductors of electricity when in these states.
- Form Crystalline Solids: Imagine a highly organized warehouse where everything is stacked neatly in an orderly fashion. Ionic compounds are similar: the positive and negative ions pack neatly together in a regular repeating pattern, forming what we call a crystal lattice. This regular arrangement gives ionic compounds their characteristic crystalline shape when solid.
So, in a nutshell, ionic compounds are like sturdy sand castles with high melting and boiling points, they act like busy highways conducting electricity when dissolved or melted, and they’re as organized as a well-maintained warehouse, forming crystalline solids!
How do metallic bonds work?
Metallic bonding can be thought of like a bustling sea filled with eager swimmers.
- The Sea of Electrons: In this scenario, the sea is actually a swarm of electrons that are free to move around. These are often called “delocalized” electrons. These electrons aren’t tied down to any one atom, instead, they float around the entire metal structure. This is a big part of what gives metals their unique properties.
- Positive Ion Cores: Now, imagine our swimmers as positive ions, the metal atoms that have lost some of their electrons to the sea. These positive ions are spread throughout the sea of electrons, much like swimmers spread out in the ocean.
The bond in a metal is a special kind of attraction between these positive ion cores and the sea of delocalized electrons. The positive ions are drawn to the negatively charged electron sea, and this keeps the structure together.
This structure is like a busy beach day: with positive ions (the swimmers) moving through a sea of delocalized electrons (the ocean). The attractive forces between the positive ions and the electron sea keeps the metal together, and allows the electrons to flow freely, giving metals their ability to conduct heat and electricity well. The metallic structure is also malleable and ductile, meaning it can be bent and stretched without breaking, similar to how water can change shape to fill different containers. So next time you see a piece of metal, imagine a sea full of tiny, energetic swimmers!
What are the properties of covalent compounds?
- Lower Melting and Boiling Points: Covalent compounds can be compared to a house of cards. While it may seem stable, a simple gust of wind (or a sneeze!) can make it collapse. This is because the forces holding the cards together (analogous to the intermolecular forces in covalent compounds) are relatively weak. So, it doesn’t require much energy (heat) to separate the molecules – thus, covalent compounds generally have lower melting and boiling points than ionic compounds.
- Poor Conductors of Electricity: Think of a covalent compound as a road full of roadblocks – it’s hard for cars to move along it freely. Similarly, in covalent compounds, there are no free ions or electrons to carry an electric current, making them poor conductors of electricity.
- Various States at Room Temperature: Imagine a mixed bag of assorted toys – some might be soft, some hard, others squishy. Just like this, covalent compounds can exist in all three states of matter at room temperature: solids, liquids, and gases. For example, water (a liquid), carbon dioxide (a gas), and sugar (a solid) are all covalent compounds.
So, to recap, covalent compounds are like a house of cards with low melting and boiling points, they’re similar to a road with roadblocks and are poor conductors of electricity, and they’re as varied as a bag of mixed toys, existing in various states at room temperature. It’s these fascinating properties that make the world of covalent compounds so diverse and interesting!
What is the difference between simple molecular structures and giant covalent structures?
Simple Molecular Structures: Think about a small family gathering. You’ve got a handful of people, all closely related, hanging out together. This is similar to a simple molecular structure. Here, we have small groups of atoms (the family members) bonded together through covalent bonds (the family ties), forming molecules. These molecules then interact with each other through weaker intermolecular forces, much like how different family groups interact at the gathering. An example of this would be a molecule of water (H2O) or methane (CH4).
Giant Covalent Structures: Now imagine a huge city with millions of people, all interconnected in a sprawling network. That’s similar to a giant covalent structure. Here, you have a huge number of atoms all covalently bonded (the city’s residents and their networks) in a repeating pattern, extending in all three dimensions. It’s not just a few atoms hanging out together, it’s a vast number of them all linked up. An example of this would be diamond or quartz, where each carbon or silicon atom is connected to others in a giant network.
The main difference between the two is the size and extent of the covalent bonding. In simple molecular structures, it’s small groups of atoms bonded together, while in giant covalent structures, it’s a huge number of atoms all interconnected. As a result, giant covalent structures typically have higher melting and boiling points due to the large amount of energy needed to break the numerous covalent bonds.
What is the structure and property of diamond and graphite?
Diamond: Picture a city where every building (carbon atom) is connected to four other buildings by strong bridges (covalent bonds). The result is a very strong and rigid structure, like a three-dimensional spider web. This network makes diamond one of the hardest known substances. It’s so hard, in fact, that it’s often used in drill bits and saw blades. Despite its strength, diamond doesn’t conduct electricity. This is because all four of each carbon atom’s electrons are used in bonding, leaving none free to carry a current.
Graphite: Now, imagine a different city, where the buildings (carbon atoms) are only connected to three others, forming flat districts (layers). These districts are separate from each other, and people (electrons) can easily move within their own district, but it’s hard for them to move between districts. The districts can slide past each other easily because the bonds between them are weak. This makes graphite soft and slippery, which is why it’s used in pencil lead. The free-moving people (electrons) within the districts allow graphite to conduct electricity, unlike diamond.
So while both diamond and graphite are made entirely of carbon atoms, the different ways those atoms are arranged results in completely different properties. It’s like how different architectural designs can create cities with very different vibes and functionalities.
What are alloys and how do they differ from pure metals?
Absolutely, let’s think about this in terms of a music band!
Pure Metals: Picture a band where every member plays exactly the same instrument. It’s harmonious and uniform, but it lacks some complexity and depth. In a similar way, pure metals consist of one type of atom arranged in a regular pattern. These atoms can slide over each other easily, which can make the metal soft and malleable, but also less strong.
Alloys: Now, imagine a band that has a variety of instruments: a guitar, a bass, drums, a saxophone. This variety makes the music richer and more interesting. An alloy is like this mixed instrument band – it’s a mixture of two or more different types of atoms (or sometimes non-metals), with the atoms of the added elements often being a different size to the original metal atoms. This disrupts the regular arrangement of the metal atoms and makes it harder for them to slide over each other, which makes the alloy harder and stronger than a pure metal.
So, in summary, while pure metals are like a band with only one type of instrument, alloys are a band with a variety of instruments, resulting in a richer and stronger material. Alloys such as steel (a mixture of iron and carbon), brass (copper and zinc), and bronze (copper and tin) are common examples of this interesting mix!
What is the difference between a dot and cross diagram and an electron shell diagram?
Dot and Cross Diagrams (Lewis Structures): These are like comic strips that specifically focus on the most important part of the story, i.e., how the characters interact. In a dot and cross diagram, only the valence (outermost) electrons of the atoms are shown, because these are the electrons involved in bonding. The atoms are usually represented by their chemical symbols, with dots and crosses used to represent electrons from different atoms. It’s a simple, focused way to show what’s happening in a chemical bond.
Electron Shell Diagrams (Bohr Models): These are more like full-page illustrations that show all the characters in the story, not just the ones interacting. In these diagrams, all of the electrons in an atom are shown, organized in energy levels or shells around the nucleus. It provides a more complete picture of the atom, but it can also be more complex and harder to draw, especially for atoms with a lot of electrons.
In summary, a dot and cross diagram (Lewis structure) is a simpler, focused diagram that shows only the valence electrons involved in bonding, while an electron shell diagram (Bohr model) is a more complete representation that shows all the electrons in an atom. Both have their uses and can help us understand different aspects of atomic structure and chemical bonding!
What is the concept of electronegativity in bonding?
Imagine you’re at a tug-of-war game, with a rope between two teams. If both teams are equally strong, the rope stays in the middle. But if one team is stronger, they pull more of the rope towards their side. In the world of atoms, electronegativity is a bit like the strength of the team in tug-of-war: it’s a measure of how strongly an atom can pull electrons towards itself when it’s in a bond with another atom.
Here’s how this works in more detail:
Electronegativity is a property of atoms that measures their tendency to attract shared electrons in a chemical bond. It’s a dimensionless quantity that was first proposed by Linus Pauling, an American chemist. On the Pauling scale, electronegativity values range from around 0.7 (the least electronegative element, francium) to 4.0 (the most electronegative element, fluorine).
Electronegativity plays a crucial role in determining the type and properties of chemical bonds:
- Nonpolar Covalent Bonds: If two atoms have the same (or very similar) electronegativities, they share the bonding electrons equally, and the bond is a nonpolar covalent bond. For example, in a molecule of oxygen (O2), the two oxygen atoms have the same electronegativity, so they share the bonding electrons equally.
- Polar Covalent Bonds: If two atoms have different electronegativities, the more electronegative atom pulls the bonding electrons towards itself more strongly, creating a polar covalent bond. This results in a charge separation, or dipole, with the more electronegative atom carrying a partial negative charge and the less electronegative atom a partial positive charge. An example is water (H2O), where the oxygen atom is more electronegative and pulls the electrons away from the hydrogen atoms, creating a polar covalent bond.
- Ionic Bonds: If the difference in electronegativity is very large, the more electronegative atom can strip electrons away from the less electronegative atom, forming an ionic bond. The resulting ions are held together by electrostatic forces. Sodium chloride (NaCl) is a common example of an ionic bond.
So, electronegativity is a key concept in chemistry that helps us understand the type of bond that forms between atoms, and the properties of the substances those bonds create!
Test Yourself
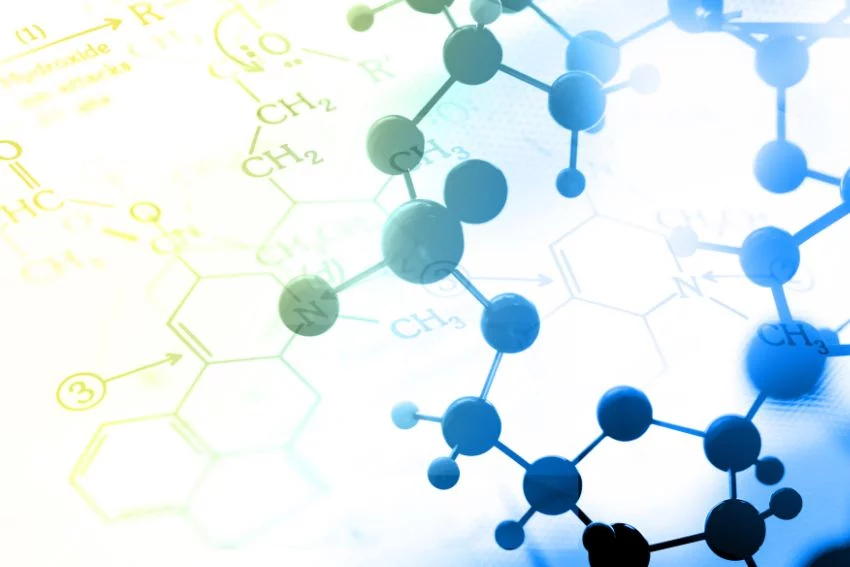
What are the three main types of chemical bonds?
- Answer: The three main types of chemical bonds are ionic bonds, covalent bonds, and metallic bonds.
What is the key difference between ionic and covalent bonding?
- Answer: In ionic bonding, electrons are transferred from one atom to another, creating ions that are then attracted to each other. In covalent bonding, electrons are shared between atoms.
What are some properties of ionic compounds?
- Answer: Ionic compounds have high melting and boiling points, conduct electricity when dissolved or melted, and form crystalline solids.
How does metallic bonding work?
- Answer: In metallic bonding, positively charged ion cores are held together in a ‘sea’ of delocalized (free-moving) electrons.
What are some properties of covalent compounds?
- Answer: Covalent compounds generally have lower melting and boiling points, are poor conductors of electricity, and can exist in all three states of matter at room temperature.
What's the difference between simple molecular structures and giant covalent structures?
- Answer: Simple molecular structures consist of small groups of atoms bonded together, while giant covalent structures consist of a large number of atoms all interconnected in a repeating pattern.
What are the structures and properties of diamond and graphite?
- Answer: Diamond is a giant covalent structure where each carbon atom is bonded to four other carbon atoms, making it very hard and a poor conductor of electricity. Graphite is also a giant covalent structure, but each carbon atom is only bonded to three others, forming layers that can slide over each other, making graphite soft, slippery, and a good conductor of electricity.
What are alloys and how do they differ from pure metals?
- Answer: Alloys are a mixture of two or more different types of atoms, usually metals. This disrupts the regular arrangement of atoms in a pure metal, making the alloy harder and stronger.
What is the difference between a dot and cross diagram and an electron shell diagram?
- Answer: A dot and cross diagram (also known as a Lewis structure) shows only the valence electrons involved in bonding, while an electron shell diagram (or Bohr model) shows all the electrons in an atom, organized in energy levels or shells around the nucleus.
What causes the high melting and boiling points in ionic compounds?
- Answer: Ionic compounds have high melting and boiling points due to the strong electrostatic forces between the positively and negatively charged ions. It takes a lot of energy (heat) to overcome these forces.
Additional Knowledge
Octet Rule
The Octet Rule is a simple chemical guideline that originates from the observation of how atoms bond together. It states that atoms tend to combine in such a way that each atom has eight electrons in its valence shell, giving it the same electron configuration as a noble gas.
So, why eight? Well, the most stable elements on the periodic table are the noble gases (like helium, neon, and argon), and they have eight electrons in their outermost shell (except for helium, which has two). This full complement of electrons makes them incredibly stable and unreactive, so it’s thought that other elements “want” to be like noble gases too.
When atoms bond together, they’re essentially trying to balance two things:
- They want to have full outer energy levels, which makes them more stable, and
- They want to have as low energy as possible, which also increases stability.
Here’s how this works with the three main types of bonds:
- Ionic Bonds: In ionic bonding, one atom (usually a metal) transfers one or more electrons to another atom (usually a non-metal). This allows both atoms to achieve a stable, full outer shell of electrons. For example, in sodium chloride (table salt), a sodium atom gives up one electron to become a positively charged ion, while a chlorine atom gains that electron to become a negatively charged ion. Both ions now have full outer shells.
- Covalent Bonds: In covalent bonding, two atoms (usually non-metals) share one or more pairs of electrons. This sharing allows each atom to count the shared electrons as part of its own full outer shell. For example, in a water molecule, each hydrogen atom shares an electron with the oxygen atom, and the oxygen atom shares one of its electrons with each hydrogen atom. This allows all three atoms to have full outer shells.
- Metallic Bonds: In metallic bonding, metal atoms pool their outer shell electrons to form a “sea” of delocalized electrons. This allows each atom to effectively have a full outer shell.
The Octet Rule is a helpful guideline for understanding chemical bonding, but it’s not without its exceptions. Some atoms can be stable with fewer or more than eight electrons in their outer shell. But for the main group elements especially, the Octet Rule often applies.
VSEPR Theory (Valence Shell Electron Pair Repulsion)
Let’s imagine a room full of people, and everyone wants to be as far from each other as possible. In the end, they’ll spread out evenly around the room. This is a bit like what happens with electron pairs around an atom.
In VSEPR Theory, the basic idea is that electron pairs (both bonding pairs that are shared with other atoms and lone pairs that aren’t shared) will arrange themselves around an atom to be as far apart as possible. Why? Because electrons are negatively charged, and like charges repel each other. This repulsion minimizes the energy of the molecule, making it more stable.
VSEPR Theory is used to predict the shape of molecules. Here’s how it works:
- Linear: If there are two regions of electron density around the central atom (like in CO2 or BeCl2), they will position themselves 180° apart, creating a linear molecule.
- Trigonal Planar: If there are three regions of electron density (like in BH3), they will spread out 120° apart in a plane, creating a trigonal planar molecule.
- Tetrahedral: If there are four regions of electron density (like in CH4), they will spread out to be as far apart as possible, creating a tetrahedral molecule.
- Trigonal Pyramidal: If there are four regions of electron density, but one of them is a lone pair (like in NH3), the molecule will have a trigonal pyramidal shape. Lone pairs take up more space than bonding pairs, so they push the other three groups slightly closer together.
- Bent or V-shaped: If there are four regions of electron density, but two of them are lone pairs (like in H2O), or if there are three regions with one lone pair (like in SO2), the molecule will have a bent or V shape.
The VSEPR theory is a useful model to predict the geometry of molecules, but like all models, it has its limitations and doesn’t perfectly predict all molecular shapes, particularly for larger, more complex molecules. Nevertheless, it’s a useful tool for understanding and predicting molecular geometries.
Pauli Exclusion Principle
The Pauli Exclusion Principle is a fundamental principle in quantum mechanics, proposed by Wolfgang Pauli in 1925.
To explain it, we first need to understand that in the quantum world, each electron in an atom is described by four quantum numbers:
- Principal quantum number (n): This defines the energy level (or shell) of the electron. It can be any positive integer (1, 2, 3, etc.).
- Azimuthal quantum number (l): This defines the shape of the orbital (the region where we’re most likely to find an electron). It can be any integer from 0 to n-1.
- Magnetic quantum number (m_l): This defines the orientation of the orbital. It can be any integer between -l and +l.
- Spin quantum number (m_s): This defines the spin of the electron. It can be either +1/2 or -1/2.
Now, the Pauli Exclusion Principle states that in an atom, no two electrons can have the same four quantum numbers. In other words, each electron in an atom has a unique address that’s defined by these four quantum numbers.
The most direct consequence of this principle is that each energy level (n) in an atom can accommodate up to 2n^2 electrons. For example, the first energy level (n=1) can hold up to 2(1)^2 = 2 electrons. The second energy level (n=2) can hold up to 2(2)^2 = 8 electrons, and so on.
Also, since the spin quantum number can only have two values (+1/2 and -1/2), each orbital can hold at most two electrons, and they must have opposite spins. This is why when you look at an electron configuration chart or the periodic table, you see each energy level divided into orbitals (s, p, d, f), each of which can hold 2, 6, 10, or 14 electrons respectively.
In summary, the Pauli Exclusion Principle is like a strict rule of occupancy for electrons in an atom: no two electrons can occupy the same state simultaneously, just as no two people can occupy the same seat at a concert.
Hund’s Rule
Hund’s Rule is another principle from quantum mechanics that helps us understand how electrons fill up the orbitals in an atom. Named after Friedrich Hund, a German physicist, it’s basically a rule of thumb for electron configurations.
Here’s what Hund’s Rule states:
- Electron Spreading before Pairing: For a given electron shell and subshell (like 2p or 3d), the electrons will fill up each orbital singly with parallel spins before they start to pair up. Think of it like people taking seats on a bus: passengers (the electrons) will generally fill up all available rows (the orbitals) before they start to sit next to each other. Just like people, electrons prefer to have their own space! So, for the 2p subshell, which has three orbitals, the first three electrons will each occupy a different orbital. Only when all three orbitals are singly occupied will the fourth, fifth, and sixth electrons pair up with the first three.
- Parallel Spins: The unpaired electrons in singly occupied orbitals have the same spin. This means that if you were to draw an arrow representing each electron’s spin, the arrows would all point the same way.
Why does this happen? It’s because electrons are not just particles, but also have properties of waves, including a property called “spin.” Electrons with the same spin are more stable when they are in different orbitals, which is why they spread out before pairing up.
In conclusion, Hund’s Rule, along with the Pauli Exclusion Principle and the Aufbau Principle (which says that electrons fill up the lowest energy levels first), allows us to predict how the electrons in an atom will be arranged, and write out the electron configuration of the atom.
Avogadro’s Law
Avogadro’s Law, named after the Italian scientist Amedeo Avogadro, is one of the gas laws and it’s all about volume and the number of particles (which could be atoms, molecules, or ions) in a gas.
Here’s what Avogadro’s Law states:
Equal volumes of gases, at the same temperature and pressure, contain an equal number of particles.
Let’s break this down:
- By equal volumes, we mean any volume – it could be one cubic centimeter, one liter, one cubic meter, etc.
- Gases can be any substance in the gaseous state.
- The same temperature and pressure are important conditions to make sure we’re comparing like with like. Just like how we might compare different brands of cookies by price per 100g, Avogadro’s Law compares different gases by volume under the same conditions.
- By equal number of particles, we mean an equal number of the smallest particles that exist separately in the gas. These could be atoms (for noble gases or gases like hydrogen or nitrogen), molecules (for gases like oxygen or carbon dioxide), or ions (in some cases).
Avogadro’s Law is very useful in chemistry because it allows us to convert between the volume of a gas and the amount of substance (usually measured in moles). This relationship is used in the ideal gas law (PV=nRT), where V is volume, n is the number of moles, and R is the ideal gas constant.
In conclusion, Avogadro’s Law is a fundamental concept in gas behavior and is vital in understanding the properties of gases and their interactions with temperature, pressure, and volume.
Metallic Bonding Model
Imagine a bustling city where people (representing electrons) move freely among skyscrapers (representing positive metal ions). This is similar to what happens in a metallic bond.
In the Metallic Bonding Model, metal atoms contribute their valence electrons to a ‘sea’ of delocalized electrons. This means that the electrons are not confined to a particular atom or a covalent bond between atoms, but are free to move throughout the entire structure of the metal.
Here’s what happens in more detail:
- Positive Metal Ions: When metal atoms come together, they lose their outermost electrons, becoming positive ions. These ions form a closely packed lattice structure.
- Sea of Delocalized Electrons: The outermost electrons that the metal atoms lose are not held by any specific atom but can move freely throughout the entire metal. This is sometimes called an “electron sea,” and it holds the positive ions together.
This metallic bonding model helps explain many properties of metals:
- Conductivity: The delocalized electrons are free to move, so they can carry electric current, making metals good conductors of electricity.
- Malleability and Ductility: The positive metal ions are held in a lattice by the electron sea, but they can slide past each other without breaking the bond. This means metals can be hammered into sheets (malleability) or drawn into wires (ductility) without breaking.
- Luster: When light hits the electron sea, it gets reflected, giving metals their shiny appearance.
- Heat Conduction: The free electrons can also transfer kinetic energy, which is why metals are good conductors of heat.
So, the Metallic Bonding Model provides a very useful way to understand the properties and behavior of metals!
Ionic Bonding Model
Imagine you’re at a dance, and one person (who’s a great dancer) has extra energy (extra electrons), and another person (who’s feeling a bit low) could really use some energy. The first person gives some energy to the second person, and they both end up feeling better. They then stick together for the rest of the dance. This is a little like what happens in an ionic bond.
In the Ionic Bonding Model, one atom donates one or more of its electrons to another atom. This creates two ions (charged atoms):
- A cation, which is positively charged because it has lost electrons. Cations are usually metal atoms.
- An anion, which is negatively charged because it has gained electrons. Anions are usually non-metal atoms.
The cation and anion are attracted to each other because of their opposite charges, and this attraction forms an ionic bond.
Here’s how this works in more detail:
- Electron Transfer: In an ionic bond, a metal atom transfers one or more of its valence electrons to a non-metal atom. This allows the metal atom to achieve a stable electron configuration (usually a full outer shell), and the non-metal atom also achieves a stable configuration by gaining the transferred electrons.
- Formation of Ions: The atom that loses electrons becomes a cation (a positively charged ion), and the atom that gains electrons becomes an anion (a negatively charged ion).
- Electrostatic Attraction: The cation and anion are attracted to each other by electrostatic forces (the attraction between positive and negative charges), and this attraction forms the ionic bond.
The Ionic Bonding Model helps explain several properties of ionic compounds:
- High Melting and Boiling Points: The electrostatic forces in an ionic bond are quite strong, so it takes a lot of energy to break them. This is why ionic compounds have high melting and boiling points.
- Solubility in Water: Water molecules are polar, which means they have a positive end and a negative end. This allows them to interact with the ions in an ionic compound and pull them apart, dissolving the compound.
- Conductivity: In a solid ionic compound, the ions are locked in place, so they can’t carry electric current. But when an ionic compound is melted or dissolved in water, the ions are free to move, so they can conduct electricity.
So, the Ionic Bonding Model gives us a useful way to understand the properties and behavior of ionic compounds!
Covalent Bonding Model
Imagine you and your friend each have a video game controller, but you both want to play a game that requires two controllers. So, you decide to share the controllers you have. This way, both of you can play the game together. This sharing is a bit like what happens in a covalent bond.
In the Covalent Bonding Model, two atoms share one or more pairs of electrons. This sharing allows both atoms to have a full outer shell of electrons (at least part of the time), making them more stable. The shared pair (or pairs) of electrons is what forms a covalent bond.
Here’s how this works in more detail:
- Shared Electrons: In a covalent bond, two non-metal atoms share one or more pairs of electrons. This allows both atoms to achieve a stable electron configuration (usually a full outer shell). The shared pair of electrons is sometimes called a “bonding pair.”
- Single, Double, or Triple Bonds: The atoms can share one, two, or three pairs of electrons, forming a single, double, or triple covalent bond. For example, in a molecule of oxygen (O2), the two oxygen atoms share two pairs of electrons, forming a double bond.
- Molecules and Molecular Compounds: Atoms joined together by covalent bonds form molecules, which can be elements (like O2 or N2) or compounds (like H2O or CO2). Substances composed of these molecules are called molecular compounds.
The Covalent Bonding Model helps explain several properties of molecular compounds:
- Lower Melting and Boiling Points (compared to ionic compounds): In a molecular compound, the forces between molecules (intermolecular forces) are generally weaker than the ionic bonds in an ionic compound, so less energy is needed to break them. This is why molecular compounds usually have lower melting and boiling points than ionic compounds.
- Poor Conductivity: Most molecular compounds do not conduct electricity, because they do not have free electrons or ions to carry electric current. However, there are exceptions like graphite, a form of carbon that can conduct electricity due to its structure.
- Diverse Physical States: Depending on the strength of their intermolecular forces, molecular compounds can exist as gases, liquids, or solids at room temperature. For example, H2O is a liquid (water), O2 is a gas, and I2 is a solid.
So, the Covalent Bonding Model gives us a helpful way to understand the properties and behavior of molecular compounds!
Conclusion
Understanding the basics of bonding, structure, and the properties of matter is essential for doing well in GCSE Chemistry. These key ideas are at the heart of many chemical reactions and the science of materials. Knowing how atoms bond, the different types of chemical structures, and how these structures affect the properties of substances helps students appreciate the wide and interesting world of chemistry.
For students looking to do their best in GCSE Chemistry, having personalised support can make a big difference. Our GCSE Chemistry tutors provide customised tutoring sessions that focus on your specific needs, helping you to understand challenging topics and develop effective study habits. Whether you need help with particular areas like covalent bonding or a thorough review of the whole syllabus, our knowledgeable tutors from top UK universities are here to help you succeed. Take advantage of this opportunity to boost your confidence and achieve your academic goals with our expert GCSE Chemistry tutors.
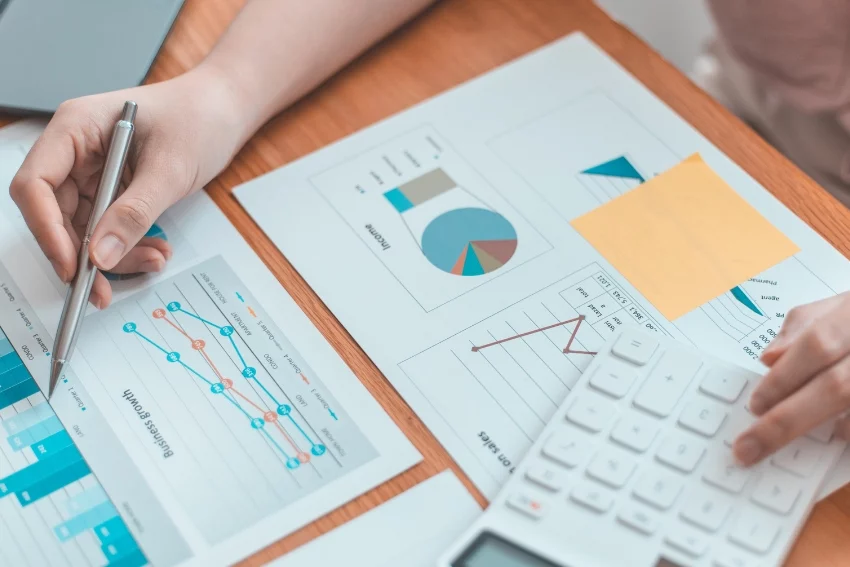