GCSE Biology – Bioenergetics
Photosynthesis: The Powerhouse of Plant Life
Photosynthesis is an incredible process that plants, algae, and some bacteria use to make their food, right out of thin air… well, almost. It’s one of nature’s most fascinating chemistry lessons and it’s happening right now in the green plants around you.
Think about this. You’ve probably heard of the saying, ‘you are what you eat.’ It’s generally used to highlight the importance of a healthy diet, but when we look at plants, it takes on a new meaning. You see, unlike us, plants don’t go grocery shopping or order a takeaway when they’re hungry. Instead, they make their food through photosynthesis.
What Happens During Photosynthesis?
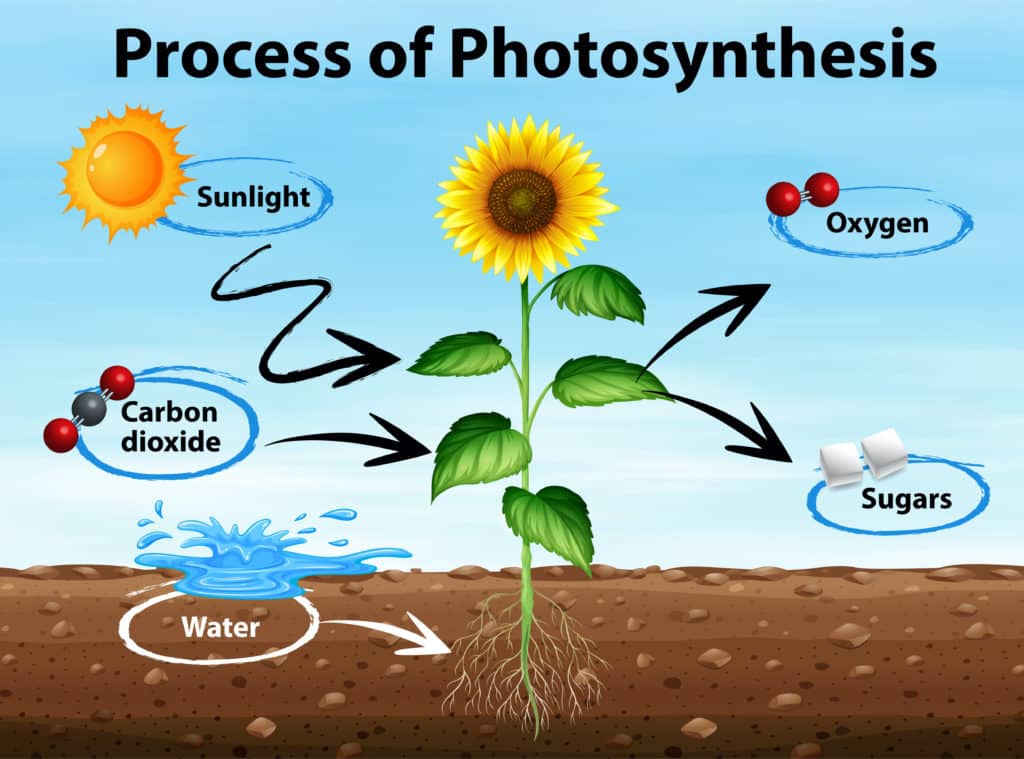
In a nutshell, plants take in carbon dioxide from the air and water from the ground, use the sunlight as a sort of power source, and convert these raw materials into glucose, a type of sugar that they use for energy. Oxygen is also produced as a by-product and is released back into the air – that’s why being around plants can feel so refreshing!
Here’s the magic recipe:
6CO2 (carbon dioxide) + 6H2O (water) + light energy -> C6H12O6 (glucose) + 6O2 (oxygen)
Now, don’t be scared off by the formula! Let’s make it simpler.
Imagine a kitchen scene. You’re the chef, and you have some ingredients – flour, water, yeast. You mix these up, put in some work kneading, and put your dough in a warm place. After some time, you’ve turned your simple ingredients into a loaf of bread – and created some gas that makes the dough rise!
Photosynthesis works in a similar way. The plant takes in water (H2O), usually absorbed by the roots from the soil, and carbon dioxide (CO2), which it gets from the air through small openings in its leaves called stomata. It then uses sunlight as its oven to combine these ingredients, creating glucose (C6H12O6) – its ‘bread’ – and oxygen (O2), which is like the gas that makes bread rise. The plant uses this glucose as a source of energy to grow, and the oxygen? That gets released back into the air, making it a win-win for us!
The Sun: The Ultimate Power Source
It’s important to know that photosynthesis only happens in the presence of light, and that’s where the Sun comes in. Just as you might need an oven or a stove to provide heat to cook your ingredients into a meal, plants need sunlight to ‘cook’ their carbon dioxide and water into glucose.
Think of sunlight as the battery that powers the whole operation. Plants have a unique way of ‘charging up.’ They contain a pigment called chlorophyll (that’s what gives plants their green color) in their leaves. This chlorophyll absorbs the sunlight and uses it as energy to kickstart the process of photosynthesis.
So next time you see a lush, green plant, remember, it’s not just a plant. It’s a mini sugar factory that uses the Sun’s energy to cook up its meals and gives us a fresh supply of oxygen in the process. And all of this happens thanks to the marvel we call photosynthesis!
Rate of Photosynthesis: Balancing the Ingredients for Life
Photosynthesis is a remarkable process that essentially allows plants to make their own food. But it’s not just a matter of the plant deciding to photosynthesise whenever it wants. The rate at which photosynthesis occurs is influenced by several key factors: light intensity, temperature, and the concentration of carbon dioxide. Let’s explore these in more detail.
Light Intensity: The Power Dial
Light is a crucial ingredient in photosynthesis. The energy from light is what powers the whole process, enabling the plant to convert carbon dioxide and water into glucose.
When light intensity increases, the rate of photosynthesis initially increases too. It’s a bit like turning up the power dial on a machine; the more power you provide, the faster it operates. However, there comes a point where the ‘machine’ is working at full capacity and can’t go any faster. In the case of photosynthesis, this is called the light saturation point. Beyond this point, increasing light intensity will not boost the rate of photosynthesis.
Temperature: Not Too Hot, Not Too Cold
Temperature plays a significant role in photosynthesis because it affects the enzymes that facilitate the process. Enzymes are like the plant’s factory workers: they help to get the job done. But these workers have their comfort zones.
If the temperature is too low, the enzymes work slowly, reducing the rate of photosynthesis. But if it gets too hot (usually above 30-40 degrees Celsius), the enzymes can be damaged or denatured, slowing photosynthesis dramatically or even stopping it.
Therefore, plants have a ‘Goldilocks’ temperature zone for optimum photosynthesis—where it’s neither too hot nor too cold, but just right.
Carbon Dioxide Concentration: The Key Ingredient
Carbon dioxide (CO2) is one of the main ingredients that a plant needs to photosynthesise. The more of this ingredient available, the faster photosynthesis can occur, up to a point.
You can think of it like baking a cake. If your recipe calls for two cups of sugar and you only have one, you can’t make the cake. Even if you have the perfect oven temperature (light intensity) and have followed the recipe exactly (temperature), you can’t get the job done without enough of a key ingredient.
Just like with light intensity, however, there is a saturation point where having more carbon dioxide won’t increase the rate of photosynthesis. That’s because the other factors (light and temperature) might become limiting, or the plant’s stomata (the pores on leaves that take in carbon dioxide) might be fully occupied.
Understanding these factors and how they interact with each other is crucial to grasp the rate of photosynthesis. In nature, plants continually adjust to changing conditions, optimising their photosynthesis process to make the most food and energy possible.
Glucose Use from Photosynthesis: Energy, Growth and Storage
In the grand scheme of photosynthesis, glucose is the pot of gold at the end of the rainbow for plants. This sugar molecule is the product of the plant’s diligent work, absorbing sunlight and sucking up carbon dioxide and water to synthesise this energy-packed molecule. But what happens to the glucose once it’s produced? How do plants use this valuable product of photosynthesis?
A Source of Energy: Fuel for the Plant
The most immediate use of glucose in a plant is as an energy source. This energy is released through a process called respiration. It’s not quite the same as animal respiration (where oxygen is used to break down food and carbon dioxide is produced); in plants, it’s more of an ‘internal combustion’ process where glucose is broken down to release energy.
Consider glucose as the petrol that keeps the plant’s engine running, enabling it to carry out various activities like growing, reproducing and repairing itself.
Building Blocks for Growth: From Glucose to Cellulose
Glucose is not only a fuel but also a building block. The glucose produced in photosynthesis can be converted into cellulose, which is used to make cell walls, particularly in rapidly growing parts of the plant such as stem and root tips.
Cellulose gives plant cells their rigid structure, allowing the plant to grow tall and reach towards the light. In a way, glucose is like the plant’s own internal manufacturing unit, producing the raw materials it needs for expansion.
Stored for Later: The Creation of Starch
Not all glucose is used immediately. Some of it is converted into starch and stored for later use. Starch is a complex carbohydrate that serves as a plant’s ‘savings account’ of energy. It’s an effective way for plants to store excess glucose because it’s insoluble, meaning it doesn’t dissolve in water and therefore doesn’t interfere with the cell’s water balance.
This stored starch can be converted back into glucose when the plant needs more energy – for example, at night, when there’s no sunlight for photosynthesis, or during winter when conditions may not be optimal for photosynthesis.
In a nutshell, glucose is the versatile outcome of photosynthesis, fuelling the plant’s activities, contributing to its growth, and being stored for future needs. Its importance to plant life – and indeed to all life on earth – cannot be overstated.
Aerobic and Anaerobic Respiration: Life’s Dual Energy Systems
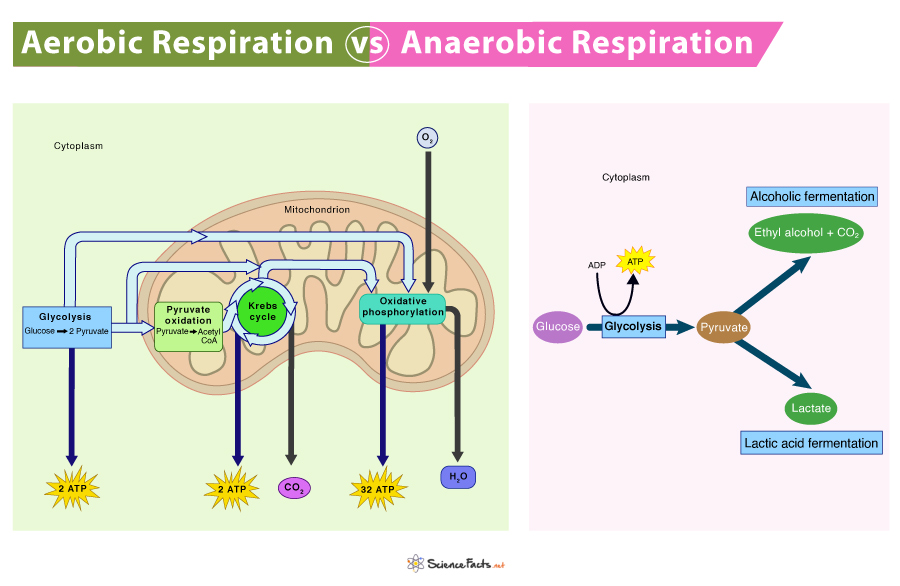
Respiration is a process by which organisms, including both animals and plants, convert nutrients into usable energy. There are two main types of respiration: aerobic and anaerobic. Understanding these two processes can give us insight into how life forms extract energy from food in both the presence and absence of oxygen.
Aerobic Respiration: Oxygen-Fuelled Energy
Aerobic respiration is the process that most of us are familiar with, occurring in both humans and plants. As the term ‘aerobic’ suggests, it’s a process that requires oxygen.
The process involves the breaking down of glucose, which is achieved through a series of chemical reactions catalysed by enzymes. In these reactions, glucose combines with oxygen resulting in carbon dioxide, water, and, most importantly, the release of energy. The overall equation for aerobic respiration looks like this:
C6H12O6 (glucose) + 6O2 (oxygen) -> 6CO2 (carbon dioxide) + 6H2O (water) + energy
This process happens continuously in the cells of plants and animals. It’s like a power plant operating 24/7 to keep the lights on and machinery running.
Anaerobic Respiration: Life without Oxygen
What happens when oxygen is scarce or not available? This is where anaerobic respiration comes in. ‘Anaerobic’ literally means ‘without air’, and as you might guess, this form of respiration does not require oxygen.
In anaerobic respiration, glucose is broken down without the aid of oxygen. This process yields less energy than aerobic respiration and also produces different by-products.
In many types of yeast and bacteria, the process of anaerobic respiration is known as fermentation, and produces ethanol and carbon dioxide as by-products:
C6H12O6 (glucose) -> 2C2H5OH (ethanol) + 2CO2 (carbon dioxide) + energy
This process is exploited in the making of bread and alcoholic beverages.
In humans and other animals during vigorous activity, when the demand for oxygen exceeds the supply, muscle cells can perform anaerobic respiration, producing lactic acid:
C6H12O6 (glucose) -> 2C3H6O3 (lactic acid) + energy
However, the build-up of lactic acid can cause muscle fatigue and needs to be broken down later when sufficient oxygen is available, a concept referred to as ‘oxygen debt’.
While anaerobic respiration is less efficient than aerobic respiration in terms of energy production, it’s an essential process for organisms living in low-oxygen environments or during times of high energy demand.
In the grand picture, both aerobic and anaerobic respiration play crucial roles in life on Earth, allowing organisms to adapt to their environment and their changing energy needs.
Response to Exercise: Body’s Adaptation to Increased Demand
Our bodies are marvels of adaptation. When we exercise, there’s a sudden demand for more energy in our muscles, and the body responds in some extraordinary ways. Understanding these changes not only gives you a deeper insight into human biology but also helps you appreciate the complexity of the systems working within us.
Heart Rate and Breathing Rate Increase
As soon as you start to exercise, your heart rate increases. This is because your muscles need more oxygen to produce energy, and your heart has to work harder to pump oxygenated blood around your body. Similarly, your breathing rate increases to take in more oxygen from the air.
Just think about it: your heart and lungs are essentially power delivery systems. When your muscles are working harder during exercise, it’s like they’re calling for more power, and your heart and lungs respond by turning up the dial.
Switching Energy Systems
At the start of exercise, your body is likely using aerobic respiration to produce energy. This process is efficient and uses oxygen to convert glucose into energy. However, as the intensity of the exercise increases, your body might not be able to supply enough oxygen to the muscles for aerobic respiration.
That’s when anaerobic respiration kicks in. This system can function without oxygen and begins to break down glucose to provide energy, producing lactic acid as a by-product. Ever felt a burning sensation in your muscles during intense exercise? That’s lactic acid build-up.
It’s important to note that anaerobic respiration doesn’t replace aerobic respiration entirely but supplements it. Think of it as a turbo boost when the main engine isn’t enough.
Recovery: Paying Back the Oxygen Debt
After you finish exercising, you’ll find yourself panting and your heart still beating faster than usual. This is because your body is in the recovery phase, or what is sometimes referred to as ‘Excess Post-exercise Oxygen Consumption’ (EPOC).
During this phase, your body is replenishing the oxygen stores in your muscles and breaking down the lactic acid produced by anaerobic respiration. Essentially, you’re ‘paying back’ the oxygen debt you accumulated during exercise.
Overall, the body’s response to exercise is a complex symphony of biological processes working together to meet the increased energy demand. It’s an incredible example of how adaptable our bodies are and how they can handle the stress of physical exertion.
Metabolism: The Body’s Chemical Symphony
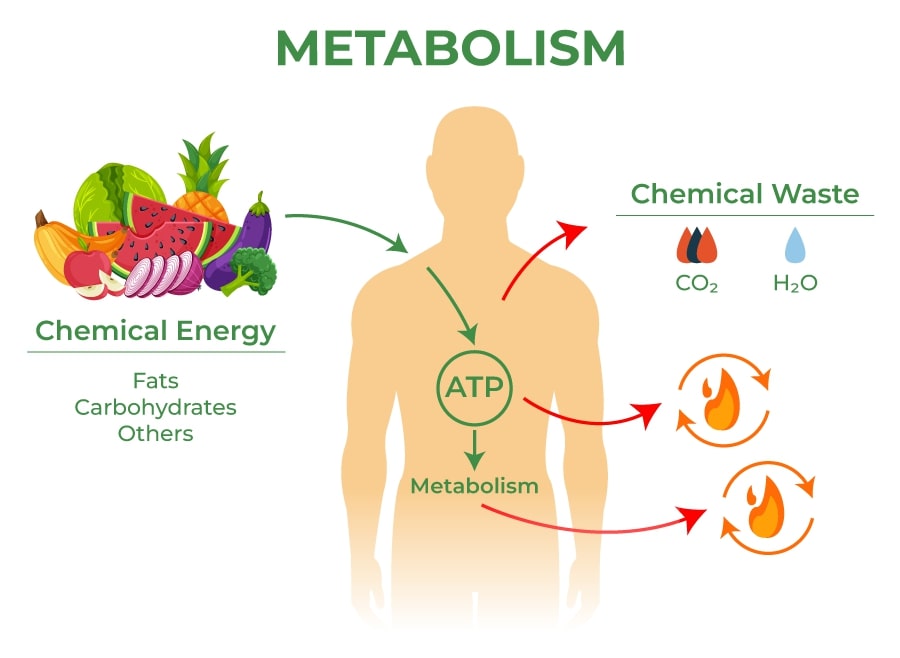
The term metabolism often conjures images of weight loss and diet in our minds. But did you know it is actually a term that describes all the chemical reactions taking place in our bodies to keep us alive and functioning? These reactions are vital for growth, reproduction, maintaining our body’s structures, and responding to our environment.
The Two Halves of Metabolism: Catabolism and Anabolism
Metabolism consists of two opposing types of activities – catabolic and anabolic.
Catabolism involves breaking down substances. During these reactions, larger molecules, such as the food we eat, are broken down into smaller ones, like amino acids, fatty acids, and sugars. In the process, energy is released. This is similar to the process of burning wood in a fire; the wood breaks down, and energy is released as heat.
Anabolism, on the other hand, is all about building up. It uses energy to construct components of cells such as proteins and nucleic acids. If catabolism is like a fire burning wood, anabolism is like building a house. It takes smaller raw materials and uses energy to create something larger and more complex.
Metabolism and Energy
The energy released during catabolic reactions, particularly in the breakdown of glucose during respiration, is stored in the molecule adenosine triphosphate (ATP). ATP is like a charged battery, holding onto energy until the cell needs it.
When the cell needs energy—for example, to contract a muscle or send a nerve impulse—ATP is broken down, releasing the stored energy and changing into adenosine diphosphate (ADP). The energy used in this process powers the cell’s activities. Later, ADP can be recharged back into ATP during catabolic reactions, ready to store more energy for future use.
Metabolic Rate
The metabolic rate is the speed at which the chemical reactions of metabolism occur in an organism. Many factors can influence metabolic rate, including body size, age, gender, physical activity, and genetics.
The term is often used in the context of weight control because the faster your metabolism, the more energy (or calories) your body needs. This is why some people can eat a lot without gaining weight (they have a high metabolism) while others put on weight easily (they have a slower metabolism).
In a nutshell, metabolism is the complex web of chemical reactions that enables life as we know it. From fuelling our cells to enabling growth and repair, understanding metabolism can give us a fascinating insight into the workings of our bodies.
Metabolism: The Body’s Biochemical Workhorse
Metabolism represents a diverse orchestra of chemical reactions in our bodies that enables us to function, grow, reproduce, heal, and respond to our environment. From breaking down the food we eat into usable energy to building up new cell components, metabolism is the biochemical
Cellular Respiration: Powering Life at a Cellular Level
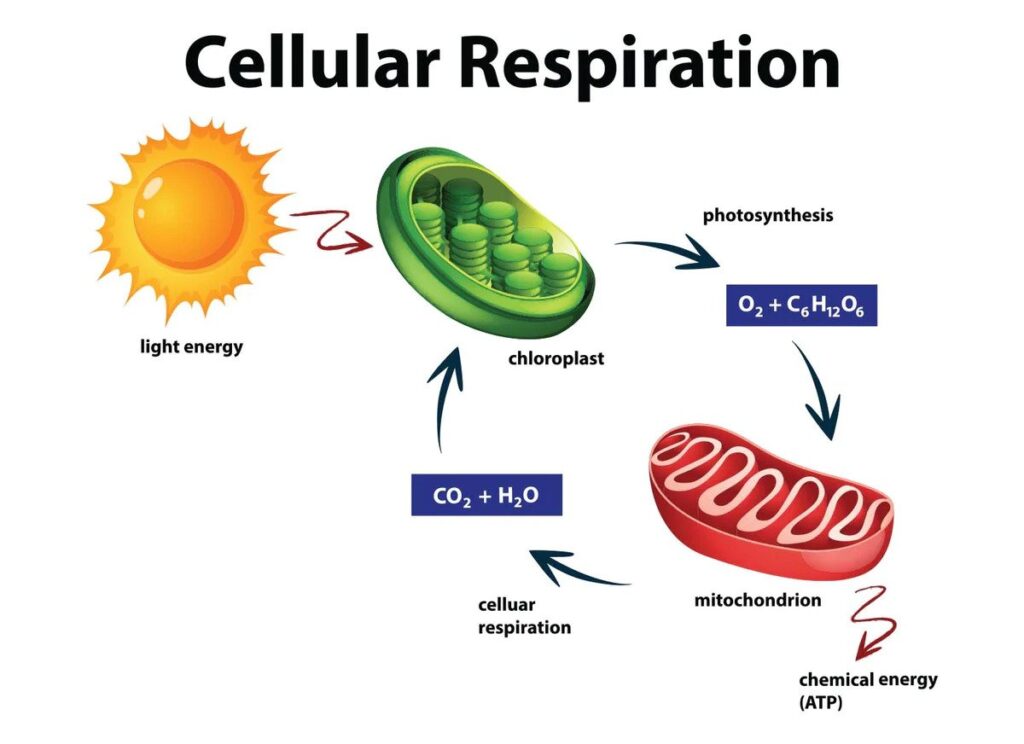
Imagine every cell in your body as a tiny engine, continuously humming along to power your life processes. This engine runs on a fuel known as ATP (Adenosine Triphosphate), a molecule that stores and releases energy as required by the cell. But where does this ATP come from? The answer is a process called cellular respiration.
What is Cellular Respiration?
Cellular respiration is a set of metabolic reactions that occur in the cells of organisms to convert biochemical energy from nutrients into ATP and then release waste products. It’s an essential process that fuels the activities of life at a cellular level.
The process primarily involves the breakdown of glucose, although fats and proteins can also be used. The glucose is oxidised, and in the process, energy is released.
The Stages of Cellular Respiration
Cellular respiration consists of three main stages: Glycolysis, the Krebs Cycle, and the Electron Transport Chain.
- Glycolysis: This is the first stage and occurs in the cell’s cytoplasm. One molecule of glucose is broken down into two molecules of pyruvate, releasing a small amount of energy. This process doesn’t require oxygen, making it an anaerobic process.
- The Krebs Cycle: Also known as the Citric Acid Cycle, this stage takes place in the mitochondria of the cell. Here, the pyruvate is further broken down, and in the process, more energy is released. This stage does require oxygen, so it’s an aerobic process.
- Electron Transport Chain (ETC): This is the final and most complex stage, occurring in the inner mitochondrial membrane. The ETC uses a series of protein complexes to transfer electrons, releasing a large amount of energy. The electrons combine with oxygen to form water, and the energy is used to produce ATP. This stage is also an aerobic process.
In total, one glucose molecule can generate up to 38 ATP molecules, though in practice, it’s often less than this due to energy loss as heat and other inefficiencies.
Cellular Respiration and Breathing
One common source of confusion is between cellular respiration and the process of breathing. While they are related, they are not the same thing. Breathing is the physical act of inhaling and exhaling, which provides the oxygen and removes the carbon dioxide needed for cellular respiration.
In essence, cellular respiration is a key biological process that allows organisms to extract energy stored in the chemical bonds of glucose and convert it into a form they can use for their cellular processes. Whether you’re running a marathon or simply reading this text, you’re using ATP generated by cellular respiration.
The Impact of Temperature and pH on Enzyme Activity
Enzymes are extraordinary biological catalysts that speed up chemical reactions in our bodies. From digestion to DNA replication, enzymes play a critical role in almost every biological process. However, their activity can be significantly affected by changes in temperature and pH.
The Effect of Temperature on Enzyme Activity
Imagine you’re trying to prepare a meal. At room temperature, butter is solid and difficult to spread, but as you heat it, it melts and becomes much easier to spread. In the same way, temperature can affect how enzymes function.
At lower temperatures, the rate of enzyme activity is typically quite slow. This is because molecules are moving slower, resulting in fewer collisions between enzymes and their substrates (the molecules enzymes act upon).
As the temperature increases, the rate of enzyme activity also increases. The molecules are moving faster, causing more frequent collisions and therefore more reactions.
However, there’s a catch. Every enzyme has an optimal temperature at which it functions best. Beyond this temperature, the enzyme starts to lose its structure in a process known as denaturation. Essentially, the enzyme begins to ‘unravel’ and loses its ability to catalyse reactions. This denaturation is typically irreversible, meaning the enzyme can’t just cool down and start working again.
The Effect of pH on Enzyme Activity
pH is a measure of how acidic or alkaline a solution is. Just like temperature, every enzyme has an optimal pH at which it works best.
Enzymes are made up of proteins, and the structure of these proteins is determined by the interactions between the amino acids that make up the protein. Changes in pH can disrupt these interactions, altering the shape of the enzyme and reducing its ability to bind to its substrate.
If the pH changes drastically from the optimum, the enzyme can denature, rendering it non-functional, similar to what happens with extreme temperatures.
Why Does This Matter?
The sensitivity of enzymes to temperature and pH has significant implications for all living organisms. Our bodies, for instance, have to carefully regulate internal temperature and pH to ensure enzymes function properly. This is why fever can be dangerous if too high – enzymes in our body start to denature.
In conclusion, enzymes are delicate yet essential biological catalysts. The conditions they work under are crucial, and even small changes in temperature or pH can dramatically impact how well they function, which in turn affects the biological processes they regulate.
Energy Transfer in Food Chains: The Journey from Sunlight to Snakes
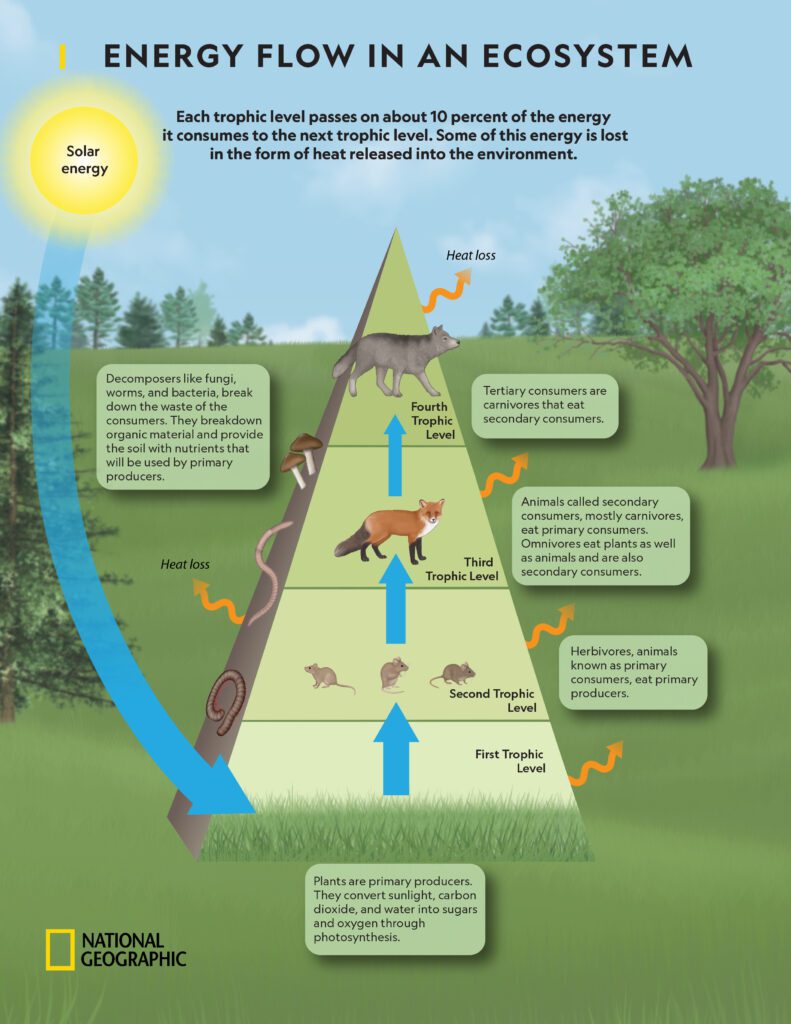
Life on Earth is dependent on the transfer of energy through various organisms in a food chain. A food chain is a linear sequence of organisms where each organism is eaten by the next one in the chain. But how does energy transfer between these organisms, and why is it important to understand? Let’s find out.
The Start of the Chain: Sunlight and Photosynthesis
The energy in a food chain almost always starts with the Sun. Green plants, or producers, harness the Sun’s energy through a process called photosynthesis. They convert sunlight, carbon dioxide, and water into glucose, which is a form of stored energy, and oxygen. This is the primary way that energy enters the food chain.
Herbivores: The Primary Consumers
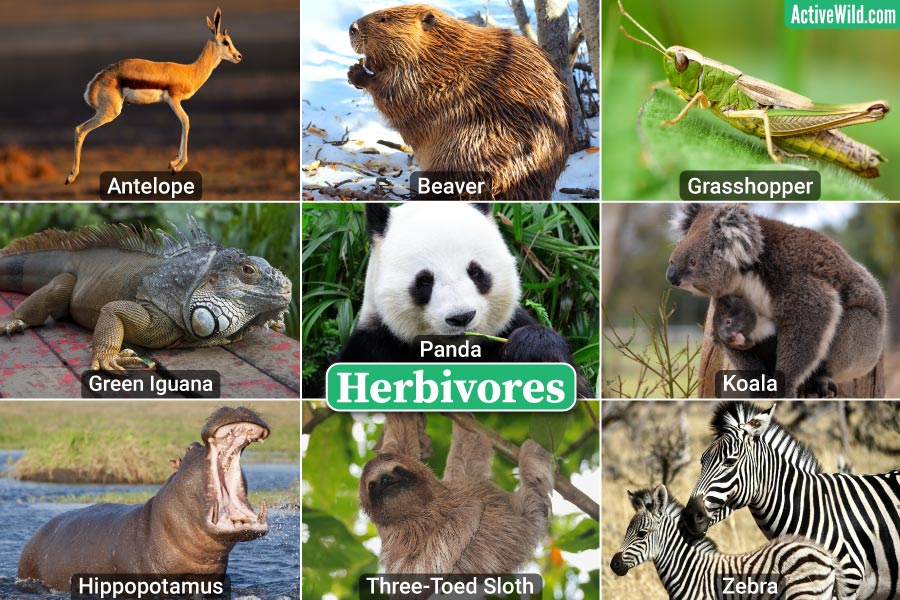
Herbivores, Source: Active Wild
The next step in the food chain are the herbivores, or primary consumers. These organisms, like rabbits or cows, eat the producers and absorb some of the energy stored in the glucose through a process called cellular respiration. However, not all the energy from the plants is transferred to the herbivore. Some is used by the plant for its own life processes, some is lost as heat, and some remains in parts of the plant that aren’t eaten.
Carnivores and Beyond: Secondary and Tertiary Consumers
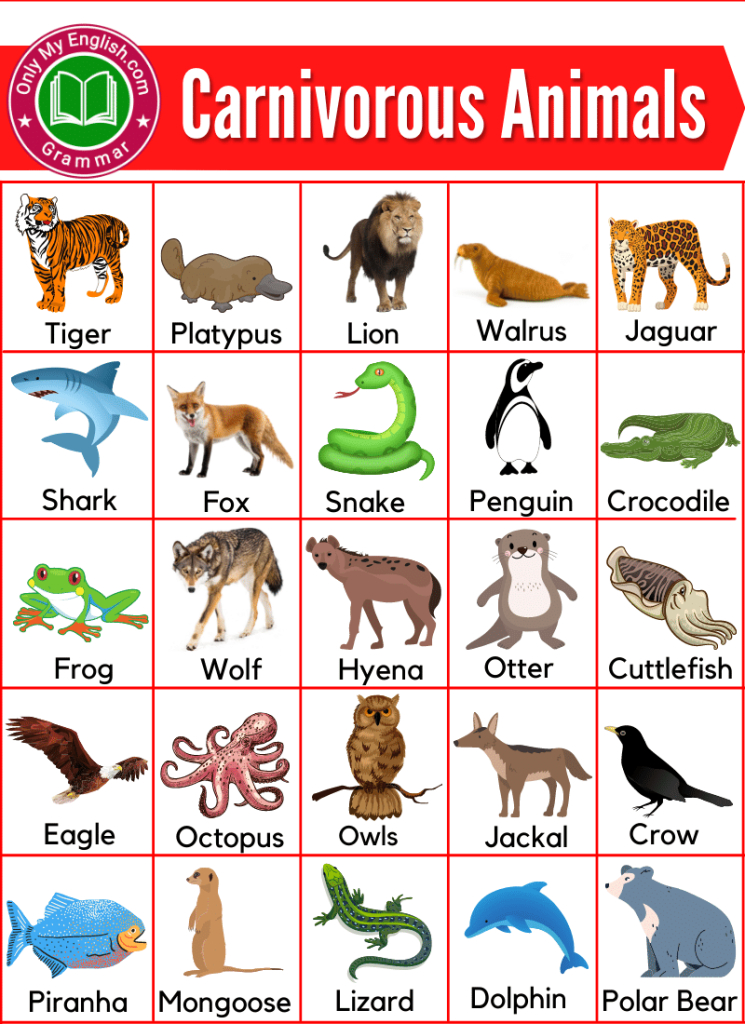
Carnivores come next in the food chain. They are secondary consumers, preying on herbivores and consuming the energy stored in their bodies. Again, not all the energy from the herbivore is passed on – some is used for the herbivore’s own life processes, lost as heat, or remains in parts of the herbivore that aren’t eaten.
The chain can continue with tertiary consumers, such as larger carnivores that eat smaller ones. The same principle applies at each stage – only a portion of the energy is passed on, with the rest being used for life processes or lost as heat.
The Rule of 10%
In general, only about 10% of the energy at each level of the food chain is passed on to the next level. This is known as the 10% rule. The remaining 90% is used by the organism or lost as heat. This is why food chains typically have only four or five levels – there’s simply not enough energy to support large numbers of organisms at higher levels.
Decomposers: The End of the Chain
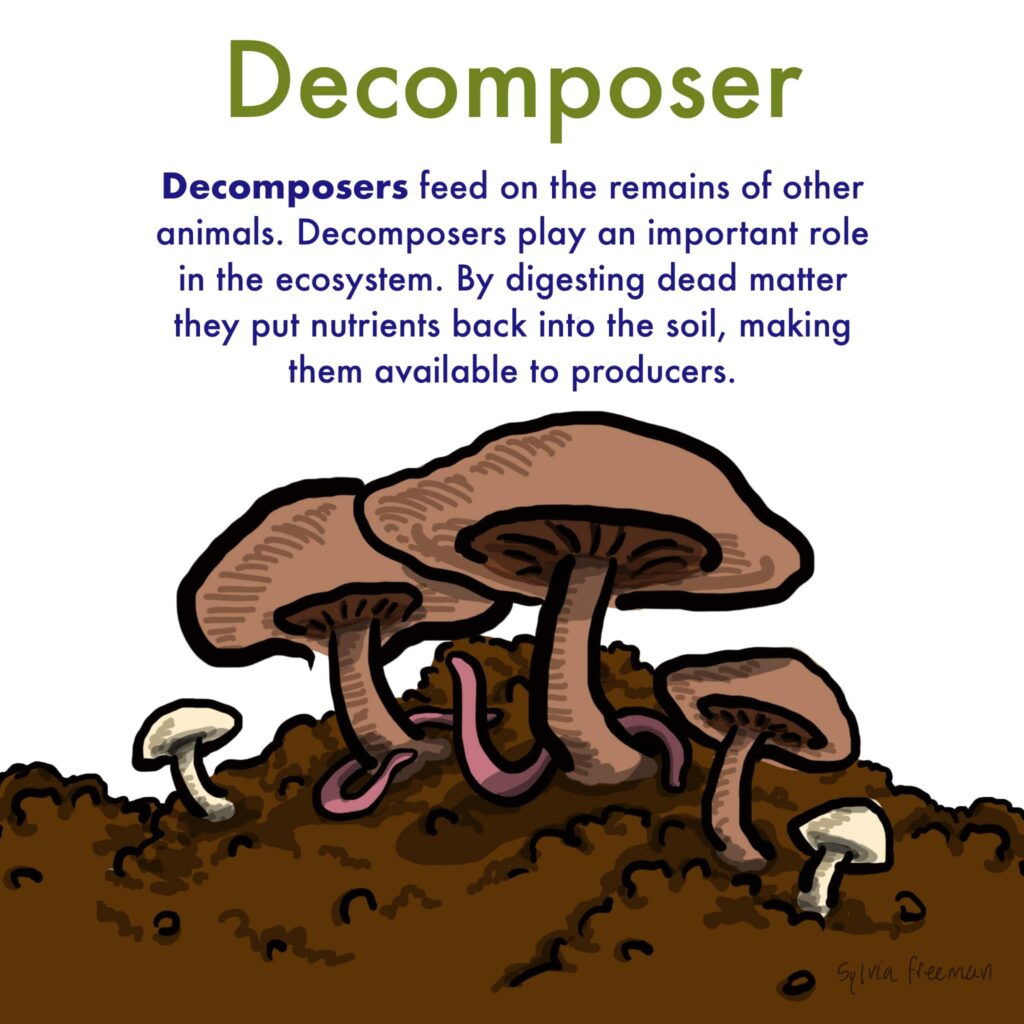
The final link in the food chain is the decomposers, organisms like bacteria and fungi that break down dead organisms and waste material. They return nutrients to the soil, which can be used by plants, thus completing the cycle and starting a new food chain.
Understanding the transfer of energy in food chains is crucial for ecology, helping us understand how organisms are interconnected and how changes in one part of the food chain can affect the whole ecosystem. Whether you’re a plant soaking up the Sun or a hawk swooping down on a mouse, you’re part of this complex, beautiful dance of energy.
Understanding ATP (Adenosine Triphosphate): The Powerhouse Molecule of Life
If we think about life on a cellular level, it’s essentially a series of chemical reactions. And those reactions need energy. But where does this energy come from? Meet ATP, or adenosine triphosphate, the primary energy currency of the cell.
What is ATP?
ATP is a complex organic molecule found in all forms of life. It consists of an adenine base, a ribose sugar, and three phosphate groups. The high-energy bonds holding the phosphate groups are the secret to ATP’s energy-storing ability.
ATP: The Energy Currency of Cells
Imagine you’re at an amusement park. Before you can enjoy any of the rides, you need to exchange your money for tokens. In the same way, your cells can’t use the energy from your food directly. They need to convert it into ATP.
When you eat, your body breaks down nutrients into smaller molecules like glucose. These molecules are then oxidised in a process known as cellular respiration, which generates ATP.
ATP: Providing Energy for Cellular Work
ATP is like a charged battery, ready to power the cell’s activities. When a cell needs energy—for instance, to move a muscle or send a nerve signal—it breaks down ATP into ADP (adenosine diphosphate) and a free phosphate group. This reaction releases energy that can be used to do work.
Once the energy has been used, the ADP and free phosphate can be recharged back into ATP during cellular respiration, ready to store more energy for future use.
ATP in a Nutshell
So, in essence, ATP acts as an intermediary, capturing energy from food and making it available for various cellular processes. It’s a system that’s elegantly simple yet vitally important. Without ATP, life as we know it would not exist.
While ATP may seem like a simple molecule, its role in sustaining life is anything but small. From supporting muscle contraction to driving the active transport of substances across cell membranes, the energy provided by ATP is the driving force behind countless biological processes. Without it, the basic functions that underpin life as we know it would simply grind to a halt.
By understanding ATP and how it works, we can appreciate the incredible complexity and beauty of life on a microscopic scale. It truly is the powerhouse molecule of life.
Understanding Fermentation: The Anaerobic Powerhouse
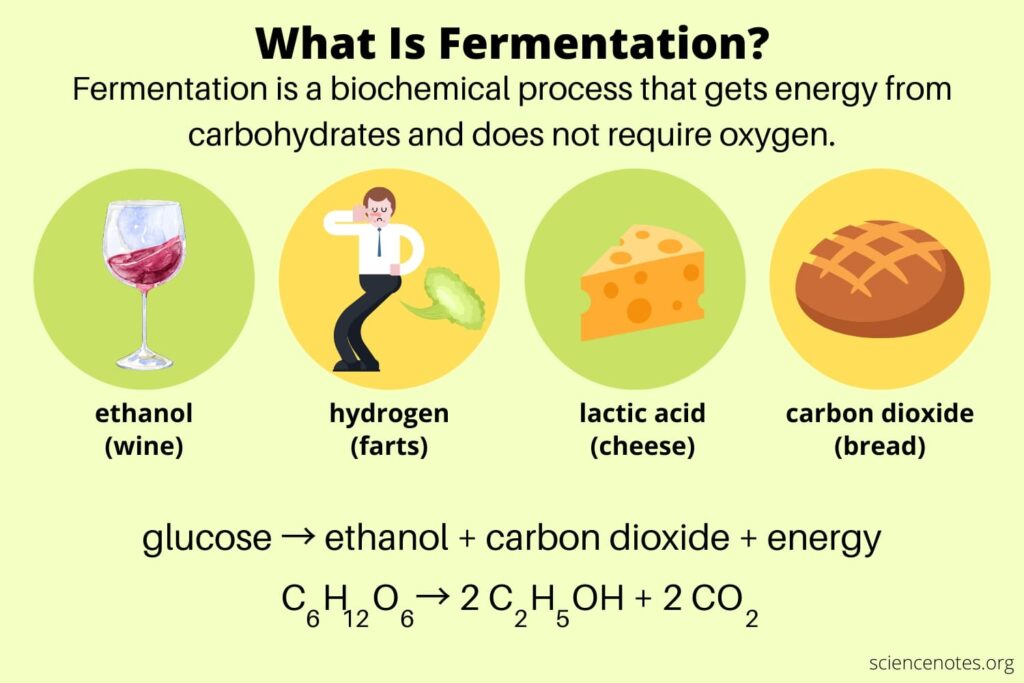
Did you know that the bubbles in your bread, the tang in your yoghurt, and even the alcohol in your wine all owe their existence to a process called fermentation? But fermentation doesn’t just make our food more enjoyable. It also plays a critical role in biology, providing organisms with energy when oxygen is scarce.
What is Fermentation?
Fermentation is a biochemical process in which an organism converts sugar into acids, gases, or alcohol in the absence of oxygen. It’s a type of anaerobic respiration, meaning it occurs without oxygen.
There are two primary types of fermentation: lactic acid fermentation and alcoholic fermentation.
Lactic Acid Fermentation
In lactic acid fermentation, glucose (or other sugars) is converted into lactic acid and energy is released. This is the process that occurs in your muscles when you’re exercising hard and your body can’t deliver oxygen to your muscles fast enough for aerobic respiration. The build-up of lactic acid is what causes the burning sensation in your muscles during intense exercise.
Interestingly, lactic acid fermentation is also the process that turns milk into yoghurt. The bacteria in the yoghurt consume the lactose (milk sugar) and produce lactic acid, which gives yoghurt its characteristic tang and texture.
Alcoholic Fermentation
Alcoholic fermentation is the process that yeast uses to produce alcohol and carbon dioxide from glucose. This is the type of fermentation that’s used to produce alcoholic beverages like beer and wine. The carbon dioxide produced during fermentation is what causes bread to rise.
Fermentation: A Survival Mechanism
While fermentation allows us to enjoy a wide range of foods and drinks, it’s also a vital survival mechanism for many organisms. When oxygen is scarce, cells can still produce energy through fermentation.
However, fermentation is less efficient than aerobic respiration. One molecule of glucose can produce up to 38 ATP molecules during aerobic respiration, while fermentation only produces 2 ATP molecules. Nonetheless, when oxygen is not available, fermentation provides a crucial lifeline to continue generating ATP and thus, sustain life.
In conclusion, understanding fermentation can enhance our appreciation of the intricate biological processes that not only aid in the survival of various organisms but also contribute to some of our favourite food and drinks. From the gym to the kitchen, fermentation is hard at work in more ways than you might realise.
Measuring the Rate of Photosynthesis: How Green Do Plants Really Grow?
If you’ve ever watched a plant grow, you’ve witnessed the results of photosynthesis. This process, in which plants convert sunlight, water, and carbon dioxide into glucose and oxygen, is the backbone of life on Earth. But how do scientists measure the rate of this vital process? Read on to find out.
The Bubble Counting Method
One simple way to measure the rate of photosynthesis is to count the number of oxygen bubbles produced by a water plant over a period of time. This experiment, often done in schools, involves placing a plant such as Elodea in a test tube filled with water and exposed to light. The plant will produce tiny bubbles of oxygen which can be counted. The higher the number of bubbles produced in a given time, the higher the rate of photosynthesis.
However, this method isn’t perfect. Not all bubbles are the same size, and it’s hard to count bubbles accurately at high rates of photosynthesis.
Using a Photosynthometer
A more accurate method involves using a piece of equipment called a photosynthometer, which measures the volume of oxygen produced by a plant over time. This machine captures the oxygen produced and allows scientists to measure it precisely, providing a more reliable estimate of the rate of photosynthesis.
Measuring the Uptake of Carbon Dioxide
Another approach to measure photosynthesis involves monitoring the uptake of carbon dioxide. Using sensors that can detect carbon dioxide concentration, scientists can measure how much of this gas is consumed by a plant over a certain period. The faster the plant uses up the carbon dioxide, the higher its rate of photosynthesis.
Factors That Affect the Rate of Photosynthesis
When measuring the rate of photosynthesis, it’s important to consider the factors that can affect it. These include light intensity, temperature, and carbon dioxide concentration. By adjusting these conditions, scientists can investigate how photosynthesis responds to different environmental conditions.
Why Do We Measure the Rate of Photosynthesis?
Understanding and measuring the rate of photosynthesis is crucial for a number of reasons. It helps scientists understand how plants respond to changes in the environment, which can be critical for crop management and addressing food security issues. It also helps in understanding global carbon cycles and the impact of human activities on these cycles.
In conclusion, photosynthesis is a vital process that supports life on Earth, and measuring its rate gives us valuable insights into plant biology and broader ecological and environmental issues.
Comparing Photosynthesis and Respiration: Two Sides of the Same Coin
At first glance, photosynthesis and respiration might seem like opposite processes. After all, one is about creating energy and the other is about using it. But if we take a closer look, we’ll see that they’re more interconnected than we think. Let’s compare these two vital biological processes and appreciate their beautiful symmetry.
The Processes
Photosynthesis is the process by which green plants, algae, and some bacteria convert light energy, usually from the Sun, into chemical energy in the form of glucose. In doing so, they consume carbon dioxide and produce oxygen. The overall equation for photosynthesis is as follows:
6CO₂ + 6H₂O + light energy → C₆H₁₂O₆ + 6O₂
Respiration, on the other hand, is the process by which cells in an organism break down glucose to release stored energy, in the form of ATP. This process consumes oxygen and produces carbon dioxide as a waste product. The overall equation for aerobic respiration is:
C₆H₁₂O₆ + 6O₂ → 6CO₂ + 6H₂O + energy (ATP)
The Comparison
At their core, both photosynthesis and respiration are about energy transformation. However, they are opposite processes:
- Energy conversion: Photosynthesis converts light energy into chemical energy (stored in glucose), while respiration converts this chemical energy into a form that cells can use (ATP).
- Gas exchange: Photosynthesis takes in carbon dioxide and releases oxygen. In contrast, respiration takes in oxygen and releases carbon dioxide.
- Location: In plants, photosynthesis mainly occurs in the chloroplasts of leaf cells, which contain the green pigment chlorophyll to absorb light. Respiration occurs in the mitochondria of all cells, be they plant or animal cells.
- Dependency on light: Photosynthesis can only occur in the presence of light, while respiration happens continuously, day or night.
Despite these differences, photosynthesis and respiration are interconnected. The glucose and oxygen produced by photosynthesis are used in respiration, while the carbon dioxide produced in respiration is used in photosynthesis. This elegant cycle allows life on Earth to flourish and is a beautiful example of how different processes in nature are interconnected.
In conclusion, while photosynthesis and respiration may seem like opposite processes, they rely on each other in a delicate and intricate balance, creating a perfect cycle that sustains life on Earth. Understanding these two processes allows us to appreciate the complex interdependencies that characterise our natural world.
Photolysis and Carbon Fixation: The Two Stages of Photosynthesis
Photosynthesis is not a single-step process but rather a complex sequence of reactions that can be divided into two main stages: the light-dependent reactions (photolysis) and the light-independent reactions, also known as the Calvin cycle or carbon fixation. Let’s break down these two stages to understand how they fit into the larger process of photosynthesis.
Photolysis: The Light-Dependent Reactions
The term “photolysis” can be broken down into “photo-“, meaning light, and “-lysis”, meaning breaking down. Thus, photolysis involves the use of light to break down molecules. In the case of photosynthesis, this occurs in the thylakoid membranes of the chloroplasts in plant cells.
During photolysis, chlorophyll (the pigment that gives plants their green colour) absorbs light energy, usually from the sun. This energy is used to split water molecules (H2O) into hydrogen ions (H+), electrons, and oxygen (O2). The oxygen is a waste product and is released into the atmosphere, which is why plants are so important for producing the oxygen that we breathe!
The hydrogen ions and electrons, on the other hand, are used to create two important molecules for the next stage of photosynthesis: ATP (adenosine triphosphate), the energy currency of the cell, and NADPH, a carrier of electrons.
Carbon Fixation: The Light-Independent Reactions (Calvin Cycle)
Once the light-dependent reactions have created ATP and NADPH, these molecules are then used in the light-independent reactions, also known as the Calvin cycle. These reactions occur in the stroma of the chloroplasts and don’t require light, which is why they can take place during the day or night.
In the Calvin cycle, carbon dioxide (CO2) from the atmosphere is “fixed” into an organic molecule in a process known as carbon fixation. The ATP and NADPH from the light-dependent reactions provide the energy and electrons needed to convert the carbon dioxide into glucose, a type of sugar that plants use for energy and as a building block for other important molecules.
In short, the two stages of photosynthesis – photolysis and carbon fixation – work together to transform light energy into chemical energy. The light-dependent reactions convert sunlight into chemical energy in the form of ATP and NADPH, and the light-independent reactions use this energy to fix carbon dioxide into glucose. This intricate process is essential for life on Earth, as it provides the energy that fuels most ecosystems and the oxygen we breathe.
Impact of Light Wavelength on Photosynthesis Rate: A Colourful Effect
We know that light is essential for photosynthesis, but did you know that not all light is created equal when it comes to this process? The colour or wavelength of light can have a significant impact on the rate of photosynthesis. Let’s explore how and why this is the case.
Understanding Light and Wavelengths
Light is made up of a spectrum of colours, each with a different wavelength. These range from the short-wavelength blue light to the long-wavelength red light, with green light in the middle. Wavelengths are important because they determine the type and amount of energy a light wave carries: shorter wavelengths (like blue) carry more energy than longer wavelengths (like red).
Photosynthetic Pigments and Light Absorption
In photosynthesis, plants use pigments like chlorophyll to absorb light and convert it into chemical energy. The main types of chlorophyll, chlorophyll-a and chlorophyll-b, absorb light most efficiently in the red and blue parts of the light spectrum and less efficiently in the green part (which is why most plants appear green—they’re reflecting green light rather than absorbing it).
How Light Wavelength Affects Photosynthesis
Because chlorophyll absorbs red and blue light most efficiently, these wavelengths drive photosynthesis more effectively than other wavelengths. That’s why if you were to plot a graph of light wavelength against the rate of photosynthesis (known as an action spectrum), you would see two peaks in the blue and red regions and a dip in the green region.
However, while blue light drives photosynthesis efficiently, it’s not as effective at penetrating water or leaf tissue as red light. This explains why many aquatic plants and deep leaf layers have accessory pigments like carotenoids and phycobilins, which can absorb light in the green and yellow ranges and pass it onto chlorophyll for photosynthesis.
Practical Applications
Understanding the effect of light wavelength on photosynthesis has practical implications. For instance, in horticulture, grow lights often use blue and red LEDs, capitalising on the wavelengths that photosynthesis uses most efficiently. Similarly, understanding light absorption in aquatic environments can help us predict the productivity of different layers of the ocean or a lake.
In conclusion, the wavelength of light plays a crucial role in photosynthesis, with red and blue light being the most effective. By understanding this, we can manipulate light conditions to maximise photosynthetic efficiency in different environments, whether it’s a farm field, a greenhouse, or an aquarium.
Oxygen Debt and Lactic Acid Fermentation: Working Hard, Breathing Harder
When we exercise vigorously, our bodies perform incredible feats of endurance and strength, but they also undergo some fascinating biological changes. One of the most intriguing is the interplay between oxygen debt and lactic acid fermentation. But what do these terms mean, and how do they affect our bodies during and after exercise? Let’s dive in.
Oxygen Debt: A Post-Exercise Payment
Also known as Excess Post-exercise Oxygen Consumption (EPOC), oxygen debt is the amount of extra oxygen our bodies need to recover after intense exercise. When we exert ourselves physically, our bodies need to produce more energy, and quickly. One of the fastest ways to do this is through aerobic respiration, which uses oxygen to convert glucose into energy. But when the demand for energy outstrips the supply of oxygen, our bodies temporarily switch to anaerobic respiration to keep up.
Anaerobic respiration is less efficient than its oxygen-using counterpart and has a significant side effect: the production of lactic acid. This build-up of lactic acid in the muscles is one of the reasons we experience muscle fatigue and soreness during and after exercise.
The “oxygen debt” comes into play after we finish exercising. Our bodies need to break down the accumulated lactic acid, repair muscle tissue, and restore energy reserves, processes that require oxygen. Hence, we continue to breathe heavily and our heart rate remains elevated for a while after we stop exercising as our bodies “repay” the oxygen debt.
Lactic Acid Fermentation: An Emergency Energy Source
Lactic acid fermentation is the process our bodies use to produce energy anaerobically, i.e., without using oxygen. During intense physical activity, when the oxygen supply to our muscles is insufficient for the demands of aerobic respiration, our bodies switch to lactic acid fermentation to keep providing energy and keep the muscles working.
In this process, glucose is broken down into two molecules of lactic acid, and in the process, two molecules of ATP (adenosine triphosphate, the body’s energy currency) are produced. While this is much less than the amount of ATP produced by aerobic respiration, it’s a faster process and can provide the extra burst of energy needed during intense exertion.
However, as a result of lactic acid fermentation, lactic acid accumulates in the muscles, contributing to muscle fatigue and soreness. This lactic acid is gradually removed from the muscles and converted back into glucose in the liver as part of the recovery process, which is facilitated by the repayment of the oxygen debt.
In conclusion, oxygen debt and lactic acid fermentation are two interlinked biological responses to intense exercise. Understanding these processes helps us appreciate the remarkable adaptability of our bodies during physical exertion and informs strategies for recovery and improved performance.
Role of Enzymes in Metabolism: The Biological Catalysts
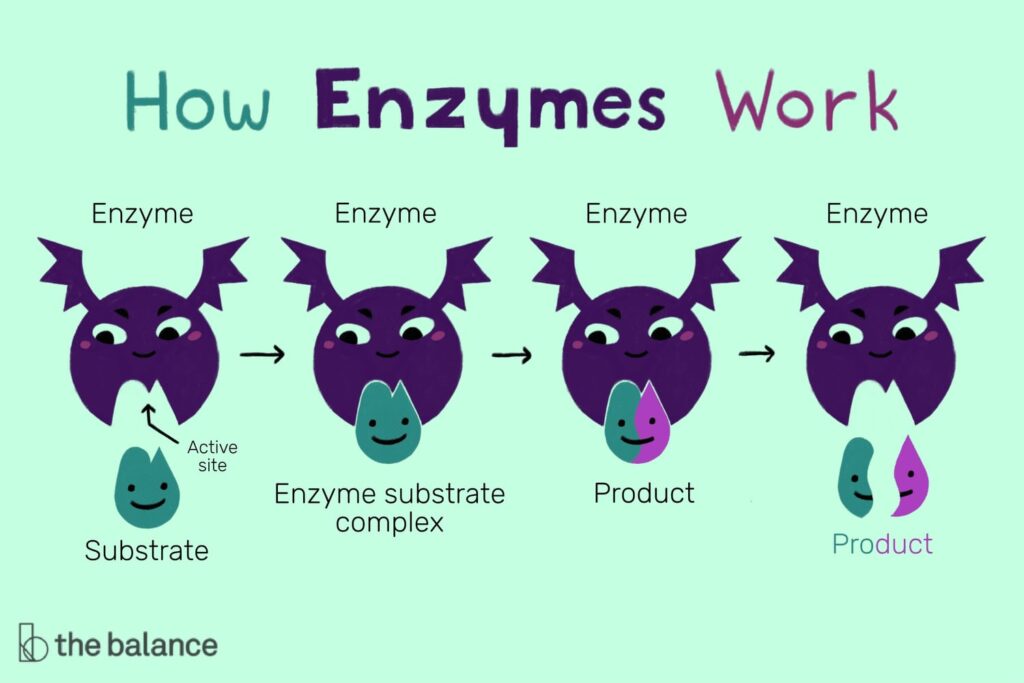
In the bustling city of a cell, countless reactions are happening every moment. These reactions, which involve building up or breaking down molecules, are what we call metabolism. And just like in any bustling city, traffic controllers are needed to keep things running smoothly. In the city of a cell, these traffic controllers are enzymes.
What are Enzymes?
Enzymes are proteins that act as biological catalysts, substances that speed up chemical reactions without being consumed by the reactions themselves. Each enzyme is specifically shaped to assist a particular reaction, fitting together with the reacting molecules (known as substrates) like a lock and key.
Enzymes in Metabolic Pathways
Metabolism involves two types of pathways: catabolic pathways, which break down complex molecules into simpler ones (like breaking down food into nutrients), and anabolic pathways, which build complex molecules from simpler ones (like synthesising proteins from amino acids).
Enzymes play a crucial role in both types of pathways, ensuring that these reactions occur fast enough to sustain life. Without enzymes, these reactions would occur too slowly to be useful.
For example, in the process of respiration, an important catabolic pathway, enzymes help break down glucose to produce energy. One such enzyme is amylase, which breaks down starch (a complex carbohydrate) into simple sugars that can be used in respiration.
In anabolic pathways, enzymes like DNA polymerase and RNA polymerase assist in the synthesis of DNA and RNA, respectively. These enzymes help join nucleotides together in the correct sequence, a critical step in the replication of genetic material and protein synthesis.
Regulation of Metabolic Reactions
Beyond just facilitating reactions, enzymes also play a role in regulating metabolism. The activity of many enzymes can be turned up or down depending on the cell’s needs. This is often achieved through feedback inhibition, where the product of a metabolic pathway inhibits an enzyme involved in its synthesis. This mechanism ensures that cells do not waste resources by producing more of a substance than they need.
The Significance of Enzymes
In conclusion, enzymes are integral to metabolism, aiding and accelerating the chemical reactions that keep cells alive and functioning. Their importance cannot be overstated: from digesting food to synthesising DNA, enzymes keep the biological world ticking over, one reaction at a time. By understanding their role, we gain insights into how life at the microscopic level is orchestrated and regulated.
What might be the consequences for a plant if it could no longer produce the enzyme Rubisco?
Rubisco is a crucial enzyme in the carbon fixation stage of photosynthesis. If a plant could no longer produce it, the plant would not be able to convert carbon dioxide into glucose. This would hinder the plant’s growth and survival.
How would a sudden decrease in temperature affect the rate of photosynthesis and why?
A sudden decrease in temperature would slow down the rate of photosynthesis because the enzymes involved in the process work best within an optimal temperature range. If the temperature drops below this range, the enzymes become less efficient, slowing the rate of photosynthesis.
Explain how the production of lactic acid during exercise can lead to muscle fatigue.
The production of lactic acid during exercise is a result of anaerobic respiration. This lactic acid builds up in the muscles, decreasing the pH and potentially interfering with the enzymes responsible for muscle contraction. This can lead to muscle fatigue.
Why do you think animals cannot carry out photosynthesis even though it could provide them with a constant source of glucose?
Animals lack the necessary structures (chloroplasts) and pigments (like chlorophyll) to carry out photosynthesis. Additionally, photosynthesis requires light, which many animals do not consistently have access to in the way plants do.
Why is oxygen debt created during intense exercise and not during regular, moderate exercise?
Oxygen debt is created during intense exercise because the rate of respiration increases to meet the demand for energy, leading to a situation where oxygen demand exceeds supply. During moderate exercise, the oxygen supply typically meets demand, so there’s no oxygen debt.
Given that ATP stores energy, why don't cells just store ATP instead of glucose or fat?
ATP is not stable for long-term energy storage. Glucose and fat molecules, on the other hand, are stable and can store a large amount of energy for their size.
In the context of bioenergetics, explain why we say that energy flows, not cycles.
Energy flows through an ecosystem, meaning it moves in one direction from producers through consumers and is eventually lost as heat. It does not cycle because it is not reused or recycled within the ecosystem.
What would happen in a food chain if the population of primary consumers drastically decreased? Explain the likely impacts on both the producers and secondary consumers.
A drastic decrease in primary consumers would likely lead to an overpopulation of producers due to reduced herbivory, while secondary consumers might face starvation due to a lack of their primary food source.
Why might an organism that lives in deep, dark ocean environments not rely on photosynthesis for its primary energy source? What might it use instead?
An organism living in deep, dark ocean environments may rely on chemosynthesis – the process of gaining energy from inorganic compounds, like sulphur, instead of photosynthesis as there is no light available for photosynthesis.
If a poison inhibits the function of enzymes involved in aerobic respiration, how might a cell be affected in terms of energy production?
If a poison inhibits the function of enzymes involved in aerobic respiration, a cell may be forced to rely more heavily on anaerobic respiration for energy. This would result in less efficient energy production and an accumulation of lactic acid or ethanol, depending on the organism.
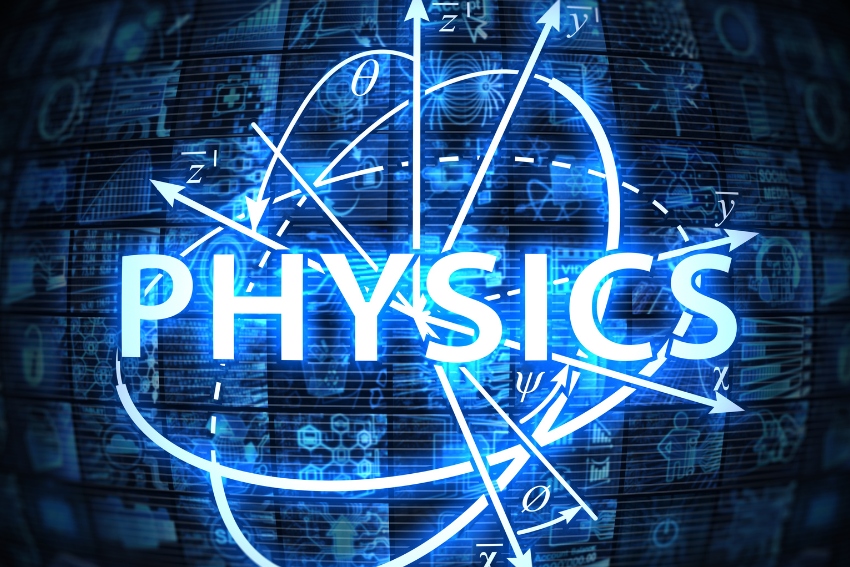